Methods, Models, and Analysis of Bacterial Adhesion
Cite this chapter.
- Itzhak Ofek 3 &
- Ronald J. Doyle 4
183 Accesses
10 Citations
It is axiomatic to consider that most living and nonliving surfaces have a tendency to be colonized by microorganisms. The importance of microbial adhesion and colonization to surfaces was not appreciated until molecular techniques were applied to analyze modes and mechanisms of cell—substratum interactions. As more and more techniques became available, new knowledge was gained that made it possible to understand the modulation of the adhesion and subsequent colonization of many microorganisms. To date, no single experimental system has been developed that can be used to adequately characterize all aspects of microbe—substratum interactions. It is therefore essential that the reliabilities, advantages, and limitations of the existing techniques be understood. Most techniques employed in the study of adhesion yield restricted amounts of information, usually about defined events in a complicated series of interactions. This chapter considers methods for the study of adhesion. Consideration is given to model systems, methods for separating adherent from nonadherent cells, controlled and uncontrolled variables in experimental design, and approaches used in analyzing adhesion data. Finally, methods related to the identification and regulation of expression of adhesins and their receptors are reviewed.
This is a preview of subscription content, log in via an institution to check access.

Access this chapter
Subscribe and save.
- Get 10 units per month
- Download Article/Chapter or eBook
- 1 Unit = 1 Article or 1 Chapter
- Cancel anytime
- Available as PDF
- Read on any device
- Instant download
- Own it forever
- Compact, lightweight edition
- Dispatched in 3 to 5 business days
- Free shipping worldwide - see info
Tax calculation will be finalised at checkout
Purchases are for personal use only
Institutional subscriptions
Unable to display preview. Download preview PDF.
Albertsson, P.A. 1958. Particle fractionation in liquid two-phase system. The composition of some phase systems and the behavior of some model particles in them. Application to the isolation of cell walls from microorganisms. Biochim. Biophys. Acta 27 : 378–395.
Article PubMed CAS Google Scholar
Allison, D.G. and I.W. Sutherland. 1984. A staining technique for attached bacteria and its correlation to extracellular carbohydrate production. J. Microbiol. Meth. 2 : 93–99.
Article CAS Google Scholar
Aronson, M., O. Medalia, L. Schon, D. Mirelman, N. Sharon, and I Ofek. 1979. Prevention of colonization of the urinary tract of mice with Escherichia coli by blocking of bacterial adherence with methyl alpha-D-mannopyranoside. J. Infect. Dis. 139 : 329–332.
Athamna, A. and I Ofek. 1988. Enzyme-linked immunosorbent assay for quantitation of attachment and ingestion stages of bacterial phagocytosis. J. Clin. Microbiol. 26 : 62–66.
PubMed CAS Google Scholar
Baselsky, V.S. and C.D. Parker. 1978. Intestinal distribution of Vibrio cholerae in orally infected infant mice: kinetics of recovery of radiolabel and viable cells. Infect. Immun. 21 : 518–525.
Google Scholar
Beachey, E.H. and I. Ofek. 1976. Epithelial cell binding of group A streptococci by lipoteichoic acid on fimbriae denuded of M proteins. J. Exp. Med. 143 : 759–771.
Brennan, M.J., J.H. Hannah and E. Leininger. 1991. Adhesion of Bordetella pertussis to sulfatides and to the GalNacß4Gal sequence found in glycosphingolipids. J. Biol. Chem. 266 : 18827–18831.
Clark, W.B., L.L. Bammann, and R.J. Gibbons. 1978. Comparative estimates of bacterial affinities and adsorption sites on hydroxyapatite surfaces. Infect. Immun. 19 : 846–853.
Costerton, J.W. 1980. Some techniques involved in study of adsorption of microorganisms to surfaces. In: Bitton, G. and K.C. Marshall (eds.), Adsorption of Microorganisms to Surfaces . John Wiley & Sons, New York, pp. 403–423.
Cowan, M.M., K.G. Taylor, and R.J. Doyle. 1986. Kinetic analysis of Streptococcus sanguis adhesion to artificial pellicle. J. Dent. Res. 65 : 1278–1283.
Cowan, M.M., K.G. Taylor, and R.J. Doyle. 1987a. Role of sialic acid in the kinetics of Streptococcus sanguis adhesion to artificial pellicle. Infect. Immun. 55 : 1552–1557.
CAS Google Scholar
Cowan, M.M., K.G. Taylor, and R.J. Doyle. 1987b. Energetics of the initial phase of adhesion of Streptococcus sanguis to hydroxylapatite. J. Bacteriol. 169 : 2995–3000.
de Man, P., B. Cedergren, S. Enerback, A.C. Larsson, H. Leffler, A.L. Lundell, B. Nilsson, and C. Svanborg-Eden. 1987. Receptor-specific agglutination tests for detection of bacteria that bind globoseries glycolipids. J. Clin. Microbiol. 25 : 401–406.
PubMed Google Scholar
Doyle, R.J. 1991. Strategies in experimental microbial adhesion research. In: Mozes, N, P.S. Handley, H.J. Busscher, and P.G. Rouxhet (eds.), Microbial Cell Surface analysis-Structural and Physiocochemical Methods . VCH Publishers, New York, pp. 293–316.
Doyle, R.J., J.D. Oakley, K.R. Murphy, D. McAlister, and K.G. Taylor. 1985. Graphical analyses of adherence data. In: Mergenhagen, S.E. and B. Rosan (eds.), Molecular Basis of Oral Microbial Adhesion . American Society for Microbiology, Washington, pp. 109–113.
Drake, D., K.G. Taylor, A.S. Bleiweis, and R.J. Doyle. 1988. Specificity of the glucanbinding lectin of Streptococcus cricetus. Infect. Immun . 56 : 1864–1872.
Duguid, J.P. and R.R. Gillies. 1957. Fimbriae and adhesive properties of dysentery bacilli. J. Pathol. Bacteriol. 74 : 397–411.
Duguid, J.P. and D.C. Old. 1980. Adhesive properties of Enterobacteriaceae . In: Beachey, E.H. (ed.), Bacterial Adherence (Receptors and Recognition , Vol. 6) . London, Chapman and Hall, pp. 184–217.
Duguid, J.P., M.R. Darekar, and D.W.F. Wheater. 1976. Fimbriae and infectivity in Salmonella typhimurium. J. Med. 11ficrobiol. 9 : 459–473.
Elbein, A.D., B.A. Sanford, M.A. Ramsey, and Y.T. Pan. 1981. Effect of inhibitors of glycoprotein biosynthesis and bacterial adhesion. In: Elliot, K., M. O’Connor, and J. Whelan (eds.), Adhesion and Microorganism Pathogenicity (Ciba Foundation Symposium 80) . Pitman Medical, London, pp. 270–282.
Ellen, R.P. and R.J. Gibbons. 1972. M protein-associated adherence of Streptococcus pyogenes to epithelial surfaces: prerequisite for virulence. Infect. Immun. 5 : 826–830.
Ellen, R.P. and R.J. Gibbons. 1973. Parameters affecting the adherence and tissue tropisms of Streptococcus pyogenes. Infect. Immun . 9 : 85–91.
Filler, S.G., L.G. Der, C.L. Mayer, P.D. Christenson, and J.E. Edwards, Jr. 1987. An enzyme-linked immunosorbent assay for quantifying adherence of Candida to human vascular endothelium. J. Infect. Dis. 156 : 561–566.
Firon, N., D. Duksin, and N. Sharon. 1985. Mannose-specific adherence of Escherichia coli to BHK cells that differ in their glycosylation patterns. FEMS Microbiol. Lett. 27 : 161–165.
Goldhar, J., A. Zilberberg, and I. Ofek. 1986. Infant mouse model of adherence and colonization of intestinal tissues by enterotoxigenic strains of Escherichia coli isolated from humans. Infect. Immun. 52 : 205–208.
Hjerten, S., J. Rosengren, and S. Pahlman. 1974. Hydrophobic interaction chromatography. The synthesis and the use of some alkyl and aryl derivatives of agarose. J. Chromatogr. 101 : 281–288.
Karlsson, J. 1986. Animal glycolipids as attachment sites for microbes. Chem. Phys. Lipids 42 : 153–172.
Klotz, I.M. 1982. Numbers of receptor sites from Scatchard graphs: facts and fantasies. Science 217 : 1247–1249.
Kluepfel, D.A. and S.G. Pueppke. 1985. Isotherm for adsorption of Agrobacterium tumefaciens to susceptible potato (Solanum tuberosum L.) tissues. Appl. Environ. Microbiol. 49 : 1351–1355.
Liljemark, W.F., C.G. Bloomquist, and L.J. Fenver. 1985. Characteristics of the adherence of oral Hemophilius species to an experimental salivary pellicle and to other oral bacteria, In: Mergenhagen, S. and B. Rosan (eds.), Molecular Basis of Oral Microbial Adhesion . American Society for Microbiology, Washington, pp. 94–102.
Ludwicka, A., L.M. Switalski, A. Lundin, G. Pulverer, and T. Wadstrom. 1985. Bioluminescent assay for measurement of bacterial attachment to polyethylene. J. Microbiol. Methods 4 : 169–177.
Mackowiak, P.A. and M. Marling-Cason. 1984. A comparative analysis of in vitro assays of bacterial adherence. J. Microbiol. Methods 2 : 147–158.
Article Google Scholar
McEachran, D.W. and R.T. Irvin. 1986. A new method for the irreversible attachment of cells or proteins to polystyrene tissue culture plates for use in the study of bacterial adhesion. J. Microbiol. Methods 5 : 99–111.
Myerthall, D.L. and T.H. Thomas. 1983. Kinetics of adherence of Actinomyces viscosus to saliva-coated silica and hydroxyapatite beads. J. Gen. Microbiol. 129 : 1387–1395.
Nesbitt, W.E., R.J. Doyle, K.G. Taylor, R.H. Staat, and R.R. Arnold. 1982. Positive cooperativity in the binding of Streptococcus sanguis to hydroxylapatite. Infect. Immun. 35 : 157–165.
Ofek, I. and E.H. Beachey. 1978. Mannose binding and epithelial cell adherence of Escherichia coli. Infect. Immun. 22 : 247–254.
Ofek, I., D. Mirelman, and N. Sharon. 1977. Adherence of Escherichia coli to human mucosal cells mediated by mannose receptors. Nature 265 : 923–625.
Ofek, I., H.S. Courtney, D.M. Schifferli, and E.H. Beachey. 1986. Enzyme-linked immunosorbent assay for adherence of bacteria to animal cells. J. Clin. Microbiol. 24 : 512–516.
Pooniah, S., S.N. Abraham, M.E. Dokter, C.D. Wall, and R.D. Endres. 1989. Mitogenic stimulation of human lymphocytes by the mannose-specific adhesin on Escherichia coli type 1 fimbriae. J. Immunol. 142 : 992–998.
Reid, G. 1989. Local and diffuse bacterial adherence on uropithelial cells. Curr. Microbiol. 18 : 93–97.
Rosan, B., R. Eifert, and E. Golub. 1985. Bacterial surfaces, salivary pellicles and plaque formation. In: Mergenhagen, S.E. and B. Rosan (eds.), Molecular Basis of Oral Microbial Adhesion . American Society for Microbiology, Washington, pp. 69–76.
Rosenberg, M. 1981. Bacterial adherence to polystyrene: a replica method of screening for bacterial hydrophobicity. Appl. Environ. Microbiol. 42 : 375–377.
Rosenberg, M., D. Gutnick, and E. Rosenberg. 1980. Adherence of bacteria to hydrocarbons: a simple method for measuring cell surface hydrophobicity. FEMS Microbiol. Leu. 9 : 29–33.
Rosenberg, M.A., E.A. Perry, D.L. Gutnick, E. Rosenberg, and I. Ofek. 1981. Adherence of Acinetobacter calcoacetius RAG-1 to human epithelial cells and to hexadecane. Infect. Immun. 33 : 29–33.
Rosenstein, I.J., D. Grady, J.M.T. Hamilton-Miller, and W. Brumfitt. 1985. Relationship between adhesion of Escherichia coli to uro-epithelial cells and the pathogenesis of urinary infection: problems in methodology and analysis. J. Med. Microbiol. 20 : 335–344.
Scaletsky, C.A., M. Silva, and R. Trabulsi, 1984. Distinctive patterns of adherence of enteropathogenic Escherichia coli to HeLa cells. Infect. Immun. 45 : 534–536.
Schadow, K.H., W.A. Simpson, and S.D. Christensen. 1988. Characteristics of adherence to plastic tissue culture plates of coagulase negative staphylococci exposed to subinhibitory concentrations of antibiotics. J. Infect. Dis. 157 : 71–77.
Smyth, C.J., P. Jonsson, E. Olsson, O. Soderlind, R. Rosengren, S. Hjerten, and T. Wadstrom. 1978. Differences in hydrophobic surface characteristics of porcine enteropathogenic Escherichia coli K88 antigen as revealed by hydrophobic interaction chromatography. Infect. Immun. 22 : 462–472.
Stanislawski, L., W.A. Simpson, D.L. Hasty, N. Sharon, E.H. Beachey, and I. Ofek. 1985. Role of fibronectin in attachment of Streptococcus pyogenes and Escherichia coli to human cell lines and isolated oral epithelial cells. Infect. Immun. 48 : 257–259.
Svanborg-Eden, C. R. Freter, L. Hagberg, R. Hull, S. Hull, H. Leffler, and G. Schoolnik. 1982. Inhibition of experimental ascending urinary tract infection by an epithelial cell-surface receptor analogue. Nature 298 : 560–562.
Valentin-Weigand, P., G.S. Chhatwal, and H. Blobel. 1987. A simple method for quantitative determination of bacterial adherence to human and animal epithelial cells. Microbiol. Immunol . 31 : 1017–1023.
Woolfson, A.D., S.P. Gorman, D.F. McCafferty, and D.S. Jones. 1987. On the statistical evaluation of adherence assays. J. Appl. Bacteriol. 63 : 147–151.
Wyatt, J.E., S.M. Poston, and W.C. Noble. 1990. Adherence of Staphylococcus aureus to cell monolayers. J. Appl. Bacteriol. 69 : 834–844.
Zilberberg, A., J. Goldhar, and I. Ofek. 1983. Adherence of enterotoxigenic Escherichia coli (ETEC) strains to mouse intestine segments analyzed by Langmuir adherence isotherms. FEMS Microbiol. Lett. 16 : 225–228.
Zilberberg, A., I. Ofek, and J. Goldhar. 1984. Affinity of adherence in vitro and colonization of mice intestine by enterotoxigenic Escherichia coli (ETEC). FEMS Microbiol. Lett. 23 : 103–106.
Download references
Author information
Authors and affiliations.
Tel-Aviv, Israel
Itzhak Ofek
Louisville, KY, USA
Ronald J. Doyle
You can also search for this author in PubMed Google Scholar
Rights and permissions
Reprints and permissions
Copyright information
© 1994 Chapman & Hall, Inc.
About this chapter
Ofek, I., Doyle, R.J. (1994). Methods, Models, and Analysis of Bacterial Adhesion. In: Bacterial Adhesion to Cells and Tissues. Springer, Boston, MA. https://doi.org/10.1007/978-1-4684-6435-1_2
Download citation
DOI : https://doi.org/10.1007/978-1-4684-6435-1_2
Publisher Name : Springer, Boston, MA
Print ISBN : 978-1-4684-6437-5
Online ISBN : 978-1-4684-6435-1
eBook Packages : Springer Book Archive
Share this chapter
Anyone you share the following link with will be able to read this content:
Sorry, a shareable link is not currently available for this article.
Provided by the Springer Nature SharedIt content-sharing initiative
- Publish with us
Policies and ethics
- Find a journal
- Track your research
An official website of the United States government
Official websites use .gov A .gov website belongs to an official government organization in the United States.
Secure .gov websites use HTTPS A lock ( Lock Locked padlock icon ) or https:// means you've safely connected to the .gov website. Share sensitive information only on official, secure websites.
- Publications
- Account settings
- Advanced Search
- Journal List
Bacterial adhesion to biomaterials: What regulates this attachment? A review
Simone kreve, andréa c dos reis.
- Author information
- Article notes
- Copyright and License information
Corresponding author at: Departamento de Materiais Dentários e Prótese, Faculdade de Odontologia de Ribeirão Preto – FORP-USP, Av. do Café, s/n 14040-904, Ribeirão Preto SP, Brazil. [email protected]
Received 2021 Mar 6; Revised 2021 May 7; Accepted 2021 May 23; Issue date 2021 Nov.
This is an open access article under the CC BY-NC-ND license (http://creativecommons.org/licenses/by-nc-nd/4.0/).
Bacterial adhesion to the surface of dental materials play a significant role in infections.
The factors that govern microbial attachment involves different types of physical-chemical interactions and biological processes.
Studying bacterial adhesion makes it possible to understand the mechanisms involved in attachment and helps in the search for technologies that promote antibacterial surfaces.
Keywords: Oral biofilms, Quorum sensing, Chemical interactions, AFM, Bacterial adhesion
Bacterial attachment to biomaterials is of great interest to the medical and dental field due to its impact on dental implants, dental prostheses, and others, leading to the need to introduce methods for biofilm control and mitigation of infections. Biofilm adhesion is a multifactorial process and involves characteristics relevant to the bacterial cell as well as biological, chemical, and physical properties relative to the surface of biomaterials. Bacteria encountered different environmental conditions during their growth and developed interspecies communication strategies, as well as various mechanisms to detect the environment and facilitate survival, such as chemical sensors or physical detection mechanisms. However, the factors that govern microbial attachment to surfaces are not yet fully understood. In order to understand how bacteria interact with surfaces, as well as to characterize the physical-chemical properties of bacteria adhesins, and to determine their interrelation with the adhesion to the substrate, in recent years new techniques of atomic force microscopy (AFM) have been developed and helped by providing quantitative results. Thus, the purpose of this review is to gather current studies about the factors that regulate microbial adhesion to surfaces in order to offer a guide to studies to obtain technologies that provide an antimicrobial surface.
1. Introduction
Biofilms play a significant role in infections associated with biomaterials [ [1] , [2] , [3] , [4] ], and when it comes to dental implants, peri-implantitis may be the main cause of failure in rehabilitation [ [4] , [5] , [6] , [7] ].
Biofilms consist of sessile bacterial communities protected by a self-produced polymer matrix that may contain proteins, carbohydrates, nucleic acids, and molecules [ 3 , [8] , [9] , [10] , [11] , [12] , [13] , [14] , [15] , [16] , [17] , [18] ]. Communities are formed by varied interactions between species and genera, which include physical cell-cell associations, known as coaggregation, interspecies signaling, secretion and renewal of antimicrobial compounds, and sharing of an extracellular matrix [ 19 ]. This matrix protects microorganisms against antimicrobial agents, facilitating the transfer of nutrients to support their survival, mediating cell-cell and cell-surface adhesion to form 3D polymeric networks, and assisting microbes to adhere and remain attached to surfaces [ 3 , 14 , 16 , [20] , [21] , [22] ]. The matrix is differently constituted depending on the specific bacterial species. The main components are polysaccharides, proteins, nucleic acids, and lipids, interacting within each other to constitute an aqueous gel-like material with proper mechanical properties and resistance to external shocks [ 23 ]. And yet, in more mature biofilms, the matrix provides physicochemical forces for biofilm adhesion to the substrate [ 8 ].
Bacterial adhesion to the surface of biomaterials involves different types of physical-chemical interactions and biological processes, with mechanisms specific to the bacteria or the substrate [ 1 , 2 , 17 ]. Bacteria promote adhesion through the development of cells that detect signals, production of extracellular polysaccharides (EPS), metabolic activity, cell viability [ 3 ] charge, hydrophobicity, cell wall stiffness, receptor-ligand binding mediated by adhesins, which are protein complexes that recognize and bind to protein receptors on the host cell surface, and appendages (pili and curli) [ 13 , 14 , 24 ]. Adhesion refers to the attachment of cells to a substrate, while cohesion is the bond between cells [ 20 , 22 ]. As well as the characteristics of bacteria, the physical-chemical parameters of the substrate [ 4 , 13 ] also regulate the adhesion and initial formation of biofilm and include surface charge, surface free energy, hydrophobicity, roughness, specific surface geometry (macro, micro, and nano), topography, and chemistry [ 1 , 13 , 15 ].
In order to prevent infections, efforts are made to introduce antimicrobial surfaces [ [25] , [26] , [27] , [28] , [29] , [30] , [31] , [32] ] including the incorporation of antibiotics [ 26 , 30 ], several antimicrobial agents [ 25 , 28 , 29 , 31 , 33 ], and micro- and nano-patterned surface technologies [ 25 , 27 , 29 , 32 ], which seek to employ antibacterial or anti-encrusting activity, based on features found in nature [ 34 , 35 ]. However, this goal has not been fully achieved yet, considering that bacteria are highly developed beings that adapt quickly to environmental signals (light, temperature, magnetic fields, and oxygen) [ 2 ], changing the constitution of their membrane, changing their surface receptors and gene expression patterns, which is the process by which the hereditary information contained in a gene, such as the DNA sequence, is used to form a functional gene product, such as proteins or RNA [ 22 ]. In addition, they have an efficient means of communication without direct physical contact, through the secretion of extracellular chemical signals, known as quorum sensing [ 11 , 13 ].
A breakthrough in the study of interactions between bacteria and substrate was the introduction of atomic force microscopy (AFM), which allows the analysis of the connection of a biofilm or a single cell with a substrate, making it possible to better explain the steps in the formation of biofilm that go beyond mass transport (when bacteria are transported to surfaces by aerosols, sedimentation or diffusion when in aqueous suspension) and initial adhesion [ 36 ]. AFM provides quantitative maps and spatial patterns of the mechanical properties of cells in a liquid environment, making it possible to assess membrane stiffness, which is a determining factor in biological response and survivability [ 13 ].
Thus, the goal of this review was to gather information on the characteristics of bacteria to understand their attachment to substrates, with a focus on surface identification and the adhesion process. The introduction of AFM as a method of quantifying the strength of adhesion is also addressed, followed by the formation of biofilm, colonizing species in teeth and implants, and communication mechanisms. Understanding the adhesion of bacteria to the substrate will make it possible to guide studies towards the search for antimicrobial surfaces.
2. Introduction and applications of atomic force microscopy (AFM) for measuring adhesion force
Bacterial adhesion to abiotic surfaces is of great interest to the scientific community because it marks the beginning of biofilm formation, and in that sense, atomic force microscopy (AFM) has opened the way for a detailed understanding of biofilms [ 2 ]. In addition to being compatible with aqueous solution environments, which is an important factor for medical research, and reaching a nanoscale resolution, AFM delivers high-resolution nanometric images [ 13 , 15 , 24 , 37 , 38 ], as well as quantitative measurements of the mechanical forces involved in cell adhesion, which can vary from 5 pN to 100 nN [ 12 ], making it possible to probe interactions at the molecular level between different species [ 2 , 19 , 37 ] ( Fig. 1 ).
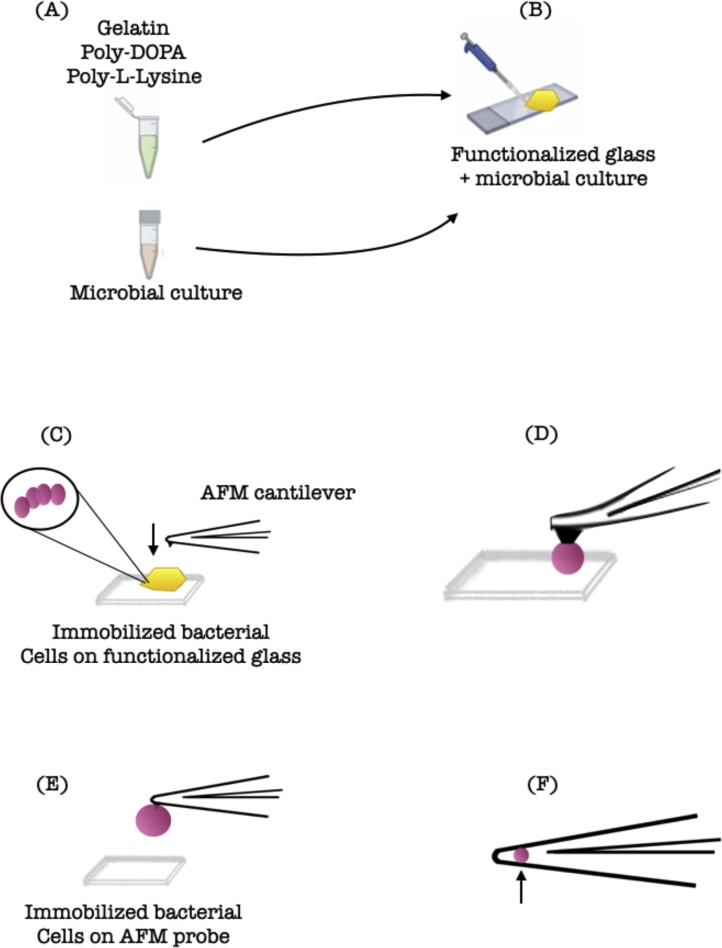
Schematic representation of single-bacterial-contact probe atomic force microscopy.
(A) The bacterial culture and the functionalizing coating are ready to be placed on the glass; (B) The glass is functionalized; (C) The bacterial cells are Immobilized on the functionalized glass; (D) The AFM probe is approached until a certain degree of indentation occurs on the cell surface; (E) Force spectra are typically captured as a cycle of tip approach and tip retraction. The tip is retracted from the surface; (F) Bacterium is attached to a tipless AFM cantilever.
The AFM consists of a nanometric tip connected to the end of a highly flexible cantilever that sweeps the sample in the x and y directions, and due to the interactions between the tip and the sample, the cantilever bends vertically (z-direction) [ 2 , 13 , 15 , 24 , 39 ], thus the force required to move the bacteria attached to a surface can be registered by a laser beam focused on the cantilever and reflected on a photodiode [ 24 , 40 ]. A piezoelectric motor maintains a defined level of deflection in the cantilever ensuring that the force applied to the sample is constant and controlled to avoid sample damage [ 40 ]. The cantilever can have a sharp tip (standard), a colloidal tip (to deliver a more defined geometry), or a functionalized tip, to probe specific chemical interactions [ 13 ]. The adhesion is recorded in newtons and determined by the force exerted by the tip on the sample [ 24 , 37 , 40 ]. The scan can be performed in constant contact, intermittent contact, or without contact [ 24 , 40 ] ( Fig. 2 ).
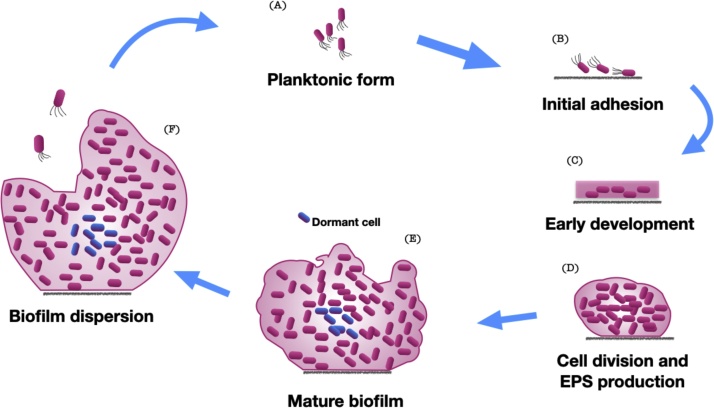
Stages of biofilm formation on (bio)materials surfaces.
(A) Planktonic form of bacteria; (B) Bacteria adhere to the surface in a dynamic process; (C) Cells aggregating and bacterial attachment becomes irreversible; (D) Bacterial form microcolonies, and start secreting extracellular polymeric substance; (E) Cells form multi-layered clusters, and maturation of the biofilm occurs; (F) Biofilm reaches a critical mass and disperses planktonic bacteria that may colonize other surfaces.
In addition, in force spectroscopy, the tip is approximated and retracted from the surface, and the force-distance (FD) curves this generates provide measurements of the physical properties of the sample, such as stiffness, elasticity, deformation, and adhesion [ 13 , 24 , 37 ]. FD-based images make it possible to map the spatial distribution of these properties in nanoscale [ 24 ]. Probe functionalization with ligands allows probing, for example, a single bacterial adhesin exposed in a live bacterium and a single protein in the extracellular matrix; as well as probing the interactions of force between a single cell and a substrate [ 24 , 37 ].
The adhesion strength of bacteria to certain surfaces is 106–108 times greater than its gravitational force, so it is not surprising that bacteria die from damage to the cell wall as a result of adhesion strength experiments [ 14 , 15 ]. Another factor observed was the existence of viscoelastic deformation of the bacterial cell wall, which was small due to the stiffness provided by the peptidoglycan layer that surrounds the membrane [ 15 ]. This layer is substantially thicker in Gram-positive bacteria than in Gram-negative bacteria. Thus, it is believed that membrane stiffness should be greater for Gram-positive cells. However, although Gram-negative cells have a much thinner layer of peptidoglycan, they have an outer membrane that acts as an extra layer and can provide additional strength [ 13 ].
Studying bacterial adhesion makes it possible to understand the mechanisms involved in attachment and helps in the search for technologies that promote antibacterial surfaces. Wang et al. [ 38 ] assessed the role of gtfB and gtfC virulence factors in the adhesion strength of Streptococcus mutans to tooth enamel and found that they are essential for adhesion. Regarding dental materials, the adhesion strength of some bacteria was measured for resinous composites, polymers used in prosthetic bases, Cobalt-Nickel-Chromium alloy, feldspar, and titanium alloys, and the adhesion strength differed according to the substrate, microorganism, and presence of salivary film [ [41] , [42] , [43] ].
The following three strength regimes were reported: (1) weak adherence, when adhesion strength is less than 1 nN. In these cases, bacteria do not realize that they are attached, and do not show any adaptive response to a substrate surface; (2) strong adherence when the adhesion strength is above 10 nN; and (3) intermediate adherence, comprising adhesion forces between 1 and 10–15 nN [ 15 ]. More recently, non-covalent protein complexes and folds with high mechanical stability have been discovered, ranging from 800 pN to 2.000 pN, found in the extracellular space, and responsible for anchoring bacteria to certain surfaces [ 39 ].
AFM has enabled advances in understanding the mechanisms of adhesion and continues to open the way to identify adhesins specific to certain bacteria [ 39 ], those involved in molecular interactions [ 24 ], as well as possible inhibitors capable of preventing adhesion and invasion of pathogens.
3. How does a bacterium know when it is near a surface?
A key element in bacteria’s adhesion process is the development of mechanisms to sense the environment, which can be by detecting chemical signals, biological molecules, or physical detection mechanisms ( Table 1 ) [ 44 ].
Mechanisms to sense environment and facilitate adhesion.
Chemical sensing depends on the presence of specific molecules, such as H + ions, antimicrobials, or biological signaling molecules [ 44 ]. The ability of bacteria to mechanically sense physical contact with substrates [ 36 , [44] , [45] , [46] ] is referred to as surface mechanosensing [ 1 , 15 ], and filamentous appendages are used by various bacteria as mechanosensors [ 12 , 17 ]. One possible mechanism for surface detection occurs when contact inhibits flagellar rotation [ 45 ]. Type IV pili, for example in P. aeruginosa , after contact with the surface, appear to inhibit retraction, which may result in a biological response [ 45 ]. Another mechanism is the deformation of the lipid membrane when in contact with surfaces, as it is loaded with environmental sensors (stress-sensitive proteins) that are activated by contact pressure, and also have mechanosensitive channels that act as interpreters of the membrane tension, and mechanical stimuli can be translated into a biological response [ 1 , 14 , 15 , 44 , 45 ]. The cell wall deformation not only increases the surface contact area but favors the physical–chemical bond [ 36 , 44 ].
Other suggested mechanosensors are the envelope protein system, such as PilY1 for P. aeruginosa [ 45 ] and NlpE-Cpx for Escherichia coli [ 12 ] and three major nucleotide-based secondary messengers, cyclic dimeric guanosine monophosphate (C-di-GMP), cyclic diadenosine monophosphate (C-di-AMP), and guanosine pentaphosphate and guanosine tetraphosphate ((p)ppGpp) [ 9 , 45 , [47] , [48] , [49] ]. In the envelope protein system, the deformation of the bacterial cell membrane is detected by the protein present in the outer membrane, which activates the protein in the inner membrane, triggering signal transduction [ 12 , 45 ]. For NlpE-Cpx, CpxA undergoes autophosphorylation and transfers its phosphate groups to the protein that regulates cytoplasmic response, CpxR, which activates the transcription of target genes [ 45 ]. The secondary messenger C-di-GMP is a master bacterial signaling molecule that controls, among other things, motility and biofilm formation. Cyclic diadenosine monophosphate C-di-GMP is involved in metabolic processes, controls essential cellular pathways such as ion transport and potassium homeostasis [ 9 , 45 , 47 , 49 ]. Bacteria use a high level of C-di-GMP to stimulate adhesin and EPS production [ 45 ]. (p)ppGpp is considered the master regulator of the stringent response, i.e., it helps bacteria survive under stressful conditions such as nutrient limitation. And further, (p)ppGpp plays a substantial role in modulating bacterial growth rate, regulation of many physiological processes such as protein biosynthesis including transcription, translation, replication, viability and virulence, acid stress response, polyphosphate metabolism, nucleotide biosynthesis, and uptake [ 48 , 50 ]. In E. coli , (p)ppGpp is produced in response to severe environmental factors (environmental stress sensors) such as lack of fatty acid, amino acid, and iron [ 51 ].
4. How does the process of surface adhesion occur?
Bacterial adhesion to a surface is a multifactorial process [ 2 ], which is affected by various factors such as duration of the bacterial exposure to surfaces, bacterial characteristics (cell wall components, appendages, and motility), nutrients, and bacterial density [ 52 ]. The cell-cell bond between two genetically distinct microorganisms is known as coaggregation, and cohesion when a cell is attached to the surface [ 24 ].
According to Straub et al., [ 1 ] the interactions involved in bacterial adhesion can be classified into three different levels, being (1) nonspecific physical-chemical interactions, (2) specific interactions, and (3) surface mechanosensing.
In non-specific physical–chemical interactions, adhesion occurs through non-covalent interactions where appendages and proteins interact with certain chemical fractions on a surface. That is, they use van der Waals forces (usually attractive), electrostatic charges (usually repulsive), or acid-base interactions (attractive or repulsive), and their characteristics are influenced by the composition of the medium, environmental pH, pressure, nutrient availability, oxygen, and surface properties [ 1 , 2 , 13 , 20 , 22 , 44 , 53 ]. Van der Waals forces have the longest range and act at distances up to 1 μm and become increasingly stronger as they get closer to the interaction surfaces [ 15 ]. Considering that the bacteria are approximately 1 μm in size, mechanosensing must be sensitive to mechanical stimuli or variations in a ∼1 μm scale [ 45 ].
In specific interactions, the adhesion mechanisms involve specific appendages capable of binding to some chemical species on certain surfaces. Coaggregation interactions are highly specific and involve the recognition of receptors in a cell type by adhesins in the partner cell. One example is when the adhesin on the cell surface of a certain bacterium recognizes a polysaccharide containing glucose, mannose, and galactose on the surface of another bacterium [ 1 , 24 ]. Streptococcus sanguinis recognizes salivary film receptors on teeth surface and forms initial bonds [ 21 ]. In addition to these receptors, S. sanguinis recognizes multiple types of fixation receptors that can bind to several components. One such example is SsaB, described as a saliva-binding protein that can mediate binding to saliva-coated hydroxyapatite via a pH-sensitive receptor [ 21 ]. Another example is Steric forces, which assist in the adhesion of some bacteria such as Pseudomonas aeruginosa , Pseudomonas putida , and E. coli , whose surfaces house a network of long chains of polysaccharides and biopolymers, responsible for generating these forces [ 2 ]. Geng et al. [ 46 ] demonstrated that in addition to detecting contact with a certain surface, E. coli can decrease breathing in response to that contact, triggering a signal [ 46 ].
In surface mechanosensing, the interaction implies the active detection of a bacterium when it comes in contact with a surface [ 1 ], and adhesion is mediated by sensory organs, such as flagella, and tension in the pili retraction. One characteristic of this adhesion is the involvement of signal transduction and response from the organism [ 1 ].
The process of adhering to a surface involves the three types of interactions (specific, nonspecific, and mechanosensing), and happens with the formation of the conditioning film, which is the base on which biofilm grows, formed by organic and inorganic particles [ 22 , 52 ]. The particles present in the fluid that bathes the surface can settle and form a part of this conditioning film. Microbes adhere to the substrates present in this layer with the aid of the appendages, by forces of attraction and/or through adhesins that interact with substances present on the surface [ 22 , 45 ]. Many types of bacteria have more than one type of adhesin [ 45 ]. In this phase, adhesion is reversible [ 13 , 36 ], especially for mobile organisms that can retain appendages, when the repulsive forces are greater than the forces of attraction, or when they do not find a suitable surface for growth, thus being able to leave the surface [ 22 ].
Irreversible adhesion progresses through the synthesis and secretion of EPS which is an essential component of the extracellular matrix [ 10 ]. EPS plays critical roles in surface adhesion, cell recognition, biofilm formation and structure, water retention, signaling, cell protection, symbiosis, nutrients, and genetic exchange [ 10 ]. The major constituents of EPS include polysaccharides, proteins, DNAs, lipids, and other polymeric compounds, and are dependent on bacterial species and environmental conditions. The EPS matrix also contains considerable amounts of proteins that are critical for adhesion and colonization, such as enzymes and protein structures like pili and fimbriae [ 8 , 10 ]. Microbial surface proteins can be generally divided into two groups: functional surface proteins like adhesins, and long-chain surface-bound macromolecules like pili [ 40 ].
The cell surface proteins of Gram-positive bacteria play crucial roles in their adhesion to abiotic and biotic surfaces [ 55 ]. In this regard, there is Sortase A, which are enzymes produced by the bacteria responsible for covalent attachment of surface-exposed proteins to the cell wall envelope of Gram-positive bacteria [ 56 ]. It plays a critical role in Gram-positive bacterial pathogenesis because it is involved in the first step of bacterial adhesion [ 51 ].
4.1. What is the role of appendages?
Appendages (pili, flagella, curli, and fimbriae) promote adhesion to surfaces through specific and nonspecific interactions [ 2 , 24 ], as described in the previous chapter. The small diameter of the appendages (flagellum, pili, fimbria) allows them to overcome repulsive electrostatic interactions, actively assisting in adhesion, as well as protein loops, DNA polysaccharides present in EPS, and patches of lipoteichoic acid that act as bonds to assist in bacterial adhesion to a surface [ 2 , 36 ].
Pili are long, flexible helical protein filaments that protrude out of bacterial cell walls. [ 2 , 55 , 57 ] They are found on the surface of Gram-negative and positive bacteria [ 55 ], and responsible for increasing initial bacterial adhesion [ 55 ], and in some organisms, they also play a role in motility within biofilms [ 24 , 45 , 58 ]. Although thin, flagella (filamentous appendix) and pili (Latin for fur) can have lengths equal to or greater than the bacteria [ 45 ]. On hydrophobic surfaces, pili strengthen adhesion by acting as nano-springs, which allows bacteria to resist high shear forces under physiological conditions [ 57 ].
The pili in Gram-negative and Gram-positive bacteria differ in how one subunit (pilins) binds to another, and how the subunits attach to the cell wall [ 55 , 57 ]. For Gram-negative bacteria, the most studied pili are type I, type IV, and P, which lengthens when subjected to a certain adhesion strength, giving rise to strength plateaus [ 12 ]. These pili, whose subunits are held together through non-covalent interactions, can withstand forces in the 250 pN range, and multiple pili often work together [ 12 , 24 ].
In Gram-positive bacteria, the pili consist of a main subunit containing one or two accessory subunits [ 57 ], which are generally linked to each other by means of covalent bonds [ 55 ], and due to such bonds, these pili behave like nano-springs, resisting forces higher than 500 pN [ 24 ]. And yet, pili can act as adhesins [ 53 ].
The presence of pili on the surface of Gram-positive pathogens is known to interact with other extracellular matrix proteins influencing bacterial adhesion and biofilm structure on biotic surfaces [ 55 ]. In a study on the function of pili on a group of non-pathogenic lactic acid bacteria, Dramé et al. [ 55 ], suggested that pili are involved in cell-cell interactions as well as the number of pili on the bacterial surface is not constant and they may influence adhesion abilities.
Most bacteria exhibit swimming filamentous mobility to generate an active self-propelled movement (which has its own means of propulsion), but even non-motile bacteria are subject to physical forces that bring them close to the surface through gravity [ 2 , 17 ]. Bacteria exhibit circular trajectories when close to surfaces, and straighter trajectories when distant [ 2 , 58 ], with this circular locomotion promoting a tendency for bacteria to be attracted to the surface, since an impelling dipole pulls the fluid present around the bacteria, and therefore directs the bacteria to the surface [ 58 ].
Flagella also play a key role in sensing and responding to surface topographies, either for swimming or adhering [ 9 , 52 ]. Some bacteria such as E. coli have multiple evenly distributed flagella responsible for controlling motility, with some groups rotating clockwise (which causes E. coli to approach the surface) and others counterclockwise (causes running) [ 9 , 52 ]. Bacteria with polar flagella, such as pseudomonads, are able to alter the swimming mode by reversing the motor [ 9 ]. This suggests that the clockwise and counterclockwise rotation of the flagellum has an important effect on cell adhesion [ 52 ]. It has been seen that flagella of certain bacteria have adhesion preferences according to surface features, with E. coli preferentially adhering in narrow line patterns [ 59 ]. And further, pili and flagella on hydrophobic surfaces can act as nano-springs, causing bacteria to resist high shear forces under physiological conditions [ 57 ].
5. Development of biofilm
The biofilm development process differs between motile and non-motile bacteria, with five stages generally involved: (1) Initial attachment of the bacteria to the surface, known as reversible adhesion; (2) Formation of a monolayer, irreversible attachment phase, which involves interaction between bacterial cells and a surface using bacterial adhesins, such as fimbriae and lipopolysaccharide; (3) Formation of multi-layered colonies, production of extracellular polymeric substances (EPS) by the resident bacterial cells; (4) biofilm maturation phase, in which bacterial cells synthesize and release signaling molecules to sense each other’s presence, leading to microcolony formation and biofilm maturation; and (5) Dispersal phase, where the bacterial cells leave the biofilms and return to an independent planktonic lifestyle ( Fig. 3 ) [ 4 , 10 , 21 , 22 , 36 ].
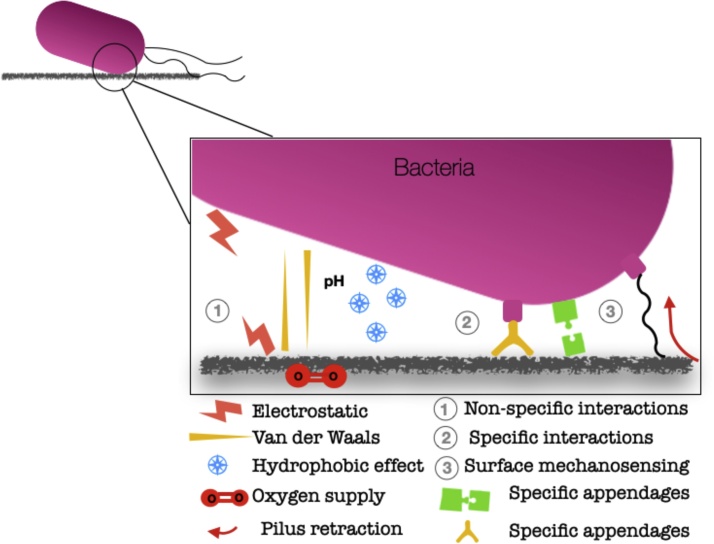
Schematic representation of bacteria proposed adhesion mechanisms.
This image was adapted from Ref. [ 1 ].
Teeth and implants exhibit a similar pattern of biofilm formation, with it taking 2–6 h after species colonization, which is faster where pellicle is present, like in freshly-cleaned natural teeth [ 19 , 54 ]. This pellicle contains proteins, salivary glycoproteins, and gingival fluid, and in implants, the formation begins 30 min after exposure in the oral cavity [ 6 , 54 ]. In this phase, biofilm is reduced due to the low albumin adsorption capacity of the film [ 54 ]. In this stage of development, the characteristics of the surface of the implants interfere with the adhesion, or not, of biofilm [ 54 ].
When an implant is implanted within the human body, they are coated with blood proteins and interstitial fluids, and this process is determined by the implant’s surface chemistry and wettability. Once implanted, bacteria use adhesins to attach to the implant surface. S. aureus and S. epidermidis have multiple biofilm attachment and formation mechanisms that contribute to their virulence in chronic infections in implants [ 53 ].
To make the film attractive, bacteria modify its surroundings. For example, early colonizers have the ability to induce conformational changes in the protein film that surrounds them [ 15 ], as well as in the production of EPS, which is another cooperative phenomenon that offers advantages in adherence to neighboring bacteria [ 15 ].
Regarding oral bacterial species, it was seen that they have undergone genetic adaptation to suit the oral cavity. This includes specialized adhesion mechanisms to allow attachment to tooth surfaces and oral mucosal tissues, metabolic coherence with the nutritional properties of saliva and gingival crevicular fluid, and the ability to interact with host defense cells [ 60 ].
An important factor in biofilm formation is hydrodynamics, which can interfere with surface detection by bacteria affecting the architecture, composition, and mechanical strength of the biofilm [ 52 ]. An example of hydrodynamics in the oral cavity is the bacterial plaque that forms on teeth that is subject to the flow of salivary and gingival crevicular fluid [ 52 ]. And yet, for S. aureus, shear flow enhances biofilm formation by increasing the production and strength of the EPS matrix [ 61 ]. And this resulting matrix plays a protective role as it allows the biofilm to recover from the mechanical challenges induced by pressure and flow, resulting in more resistant, compressible biofilms that favor bacterial growth [ 52 ].
The matrix also provides limited molecular dispersion, makes the biofilm a protected environment, facilitates communication, metabolite exchange, and protection from external threats [ 23 ]. The extracellular matrix, made of polysaccharides, proteins, and extracellular DNA, can prevent some antibiotics from successfully penetrating cells, inducing antibiotic tolerance [ 62 ].
5.1. Are initial colonizers that adhere to teeth the same as those that adhere to implant surfaces?
Initial colonizers make the attachment of successive organisms possible, so that biofilm is formed. In this sense, inter-bacterial coaggregation is well established for Streptococcus , Actinomyces , and Veillonella [ 63 ]. Enamel contains Streptococcus spp., S. sanguinis , Streptococcus mitis , and Streptococcus oralis [ 54 , 64 ] which adhere to the acquired film components through selective binding of the adhesin receptor [ 19 , 65 ]. Actinomyces , Gemella , Neisseria , and Veillonella can also be found. During the first 48 h of growth, there is a change in the balance of the first colonizers, with an increase in the presence of Streptococcus spp. and a decline in the amount of Actinomyces spp. [ 19 ]. If gingivitis is established, there is an increase in Fusobacterium , Lachnospiraceae , Lautropia, and Prevotella species [ 65 , 66 ], whereas, in periodontitis, P. gingivalis , T. forsythia , Prevotella intermedia , and T. denticola are observed [ 19 , 66 ].
Gram-negative oral bacteria produce a variety of adhesins that contribute to both polymicrobial biofilm formation and host cell interactions. For example, long (FimA) and short (MfaI) pili of P. gingivalis are involved in host cell coaggregation and adhesion [ 8 ].
One microorganism considered to be a key factor in the development of oral biofilm for helping in the attachment of successive organisms is S. sanguinis . It is an optional Gram-positive anaerobic [ 4 ], which uses a wide range of carbohydrate sources for survival [ 21 ] and is abundant in supragingival and subgingival plaque [ 21 ]. It is usually reported as non-motile, although S. sanguinis produces short type IV pili that do not confer motility but are important for adhesion to host cells [ 8 ].
The main secondary colonizers include species of Actinomyces , S. mutans , and S. sobrinus . Some bacteria, like Fusobacterium nucleatum , can bind to the initial and secondary colonizers, multiplying and coaggregating with other species [ 54 ].
Bacteria that infect implants usually present as bacterial aggregates involved in an abundant EPS matrix [ 53 ]. The microbiota of healthy implants is composed of Gram-positive rod cells and cocci [ 6 , 54 ] and in peri-implant infections, there is the presence of Gram-negative bacteria such as Veillonella sp. and spirochete including Treponema denticola [ 54 ]. It has recently been demonstrated that the peri-implantitis microbiome differs from the periodontitis microbiome [ 5 ]. According to DAUBERT & WEINSTEIN [ 6 ], there is still no consensus on the specific microbial profile associated with peri-implant diseases. However, it is suggested that factors such as surface roughness, free energy, chemistry, and titanium purity, as well as the patient’s periodontal condition, might influence the microbiome [ 6 ].
Three key factors can be considered important for the microbiota shift during periodontal disease. First is the presence of organisms that subvert the inflammatory response by triggering a state of dysbiosis and inflammation, and these influence a change in the entire bacterial population. The second is an elevated commensal microbial population activity is observed and the third is the ability of the oral microbial population to form biofilms enabling multiple species to exist [ 65 ]. And yet, in addition to these factors, there are the individual characteristics of the oral cavity of each person, and that influence the bacterial environment of the mouth, such as diet and consequently the availability of nutrients, host immune response, saliva pH, hydrogen ion concentration, and hygiene [ 64 ].
A change from a healthy peri-implant groove to a peri-implant pouch is associated with an increased presence of cocci, motile bacilli, and spirochetes [ 67 ]. Do Nascimento et al. [ 68 ] have found relevant microbial counts containing the species T. denticola , T. forsythia , P. gingivalis , F. nucleatum , P. intermedia and, A. actinomycetemcomitans , but with significant differences depending on the material used for the implant and the collection site [ 68 ]. The genus Eubacterium , Staphylococcus aureus , and Filifactor alocis were also found in peri-implantitis lesions [ 5 ].
6. Quorum sensing
Bacteria have a communication mechanism that allows them to produce, detect and respond to signals produced by other microorganisms of the same or different species [ 11 , 13 , 18 , 22 , 36 , 54 , 69 ]. This is called quorum sensing and consists of an enzyme that catalyzes the synthesis of chemical signals, and a receptor that binds to the signal and induces the expression of genes responsible for various physiological mechanisms, such as sporulation, biofilm production, conjugation, and motility, in addition to virulence factors, such as proteases, toxins, and adhesins [ 11 , 18 , 62 ]. Biofilm formation is directly regulated by this quorum sensing activity, with the matrix optimizing and detecting the signaling [ 8 , 54 ]. Bacterial quorum sensing depends on a series of events such as signal production, signal dissemination, signal receptors, signal detection, gene expression, and signaling response [ 10 ] ( Table 2 ).
Quorum sensing (QS) system in Gram-negative and Gram-positive bacteria.
There are at least three classes of quorum detection mechanisms, also called inducers: (1) LuxI/LuxR detection in Gram-negative species with acyl-homoserine lactone (AHL) signals, with these Lux proteins producing a specific AHL for each bacterial species, and variations in AHL occurring according to the length of the carbon chain [ 11 , 20 , 70 ]; (2) detection of peptides produced by Gram-positive bacteria (AIPs), which are species- and strain-specific; and (3) Lux-S encoded autoinducer-2 (AI-2), which is another class of signaling molecules and can be found in Gram-positive and Gram-negative bacteria [ 11 , 18 , 22 , 51 , 54 , 62 , 69 , 70 ]. Because it is widely used for interspecies communication, AI-2 is considered a signal for universal communication between different species [ 8 , 10 , 11 ]. A wide variety of other signaling molecules have also been identified and include fatty acids used by Xanthomonas spp., Burkholderia spp., Xylella spp. ketones, epinephrine, norepinephrine, and AI-3, or quinolones [ 62 ] ( Table 3 ).
Main strategies to interrupt biofilms.
Some bacteria have their own quorum-sensing signaling systems. Signaling among oral Streptococcus spp. strains involve peptides, such as the competence-stimulating peptide or the X-inducing peptide of S. mutans [ 8 ]. Pseudomonas aeruginosa employs a quorum-sensing system via the Pseudomonas quinolone signal (PQS) [ 51 , 71 ].
The quorum allows bacteria to coexist in a community and express phenotypes that are advantageous for the group and ensure survival [ 11 , 18 ], since this system begins with the production and release of autoinducers into the environment, whether by the pathogen or the resident microbiota. It has been found that communication is effective among Gram-negative bacteria that are up to 78 μm apart [ 18 ]. However, the most effective distances for communication occur between 4 and 5 μm [ 15 , 18 ].
Quorum sensing systems can be expressed in different bacterial pathogens, and some examples occur in P. aeruginosa , where the quorum sensor plays a role in the generation of extracellular DNA, controlling the production of rhamnolipid biosurfactant, and of siderophores such as pyoverdine and pyochelin, which are important for biofilm formation [ 15 , 16 ]. Another example is hydrogen peroxide (H 2 O 2 ) produced in vitro by some oral streptococci, and when present in sublethal concentrations, they trigger signaling responses in C. albicans [ 19 ].
Due to the need to seek antimicrobial therapies alternative to antibiotics, quorum-quenching is studied as an alternative to inhibit biofilm formation by inhibiting or interrupting quorum detection [ 15 , 20 , 69 , 70 ]. Interferences with quorum sensing are called quorum quenching, a natural phenomenon where an enzyme degrades AHL signals and leads to the disruption of the quorum-sensing signal [ 51 , 62 ]. There are some bacteria called non-conformists, which represent a subpopulation that does not obey quorum detection commands. They act by interfering with the binding of the receptor signal, decreasing its concentration, or inhibiting certain enzymes capable of degrading signaling molecules [ 20 , 69 ]. In the environment, there are many compounds that affect communication, and, based on their molecular weight and chemical composition, these compounds can be macromolecular enzymes or microparticulate quorum-quenching inhibitors [ 20 ]. Some examples may be natural products, such as polyphenols isolated from tea or honey, ajoene from garlic, eugenol from clove, or many others produced by marine organisms and fungi [ 62 ]. And yet, products arising from bacteria, plant, and animal derivatives are also studied, which include enzymes such as AHL Acylases, AHL Lactonases, and Oxidoreductases, as well as alternative materials that include nano-molecules, and nano- and micro-composites, based for example, on Ag or ZnO, or chemicals with small molecules, such as 5-Fluorouracil (5-FU) and halogenated furanones [ 11 , 20 , 70 ].
7. Strategies for interrupting biofilm formation
Some approaches are studied to disrupt biofilm formation or to prevent its diffusion. Some strategies are based on materials engineering, where anti-adhesive surfaces are created or antibacterial additives are incorporated into substrates [ 52 ]. Other areas research ways to act directly on the bacteria, either by inhibiting the quorum sensing system, preventing the formation of extracellular matrix, and, among others, inhibiting the signaling pathways of secondary messengers [ 51 ].
Regarding the material’s topography, it was seen that characteristics concerning the size, shape, and distribution of roughness patterns affect both the attachment and biofilm formation of different bacterial strains on various substrates. Bacterial adhesion decreases as the size of the topographical pattern get smaller [ [72] , [73] , [74] ], and in this sense, topographies on a micron-scale mainly affect bacterial fixation, whereas topographies on a nanoscale may have bactericidal effects [ 9 ].
Nanometric surfaces were inspired by antibacterial activities seen in nature, such as those that occur in lotuses, cicadae, sharks, butterflies, and among others, dragonfly wings [ 35 , 52 ]. Recent studies [ 75 , 76 ] attempt to replicate these patterns by developing surfaces for future application in the biomedical field, such as PMMA surfaces designed as shark skin-patterned [ 75 ], and zirconium-based bulk metallic glasses [ 76 ]. However, this area of research is still in its infancy and further studies are needed before these materials can be used.
Regarding the incorporation of antimicrobial agents to biomaterials, specifically in dentistry, different nanoparticles and agents have been incorporated in order to prevent biofilm formation without changing the physicochemical and mechanical properties.
Nanometric materials have increased antimicrobial activity due to their larger surface area and chemical reactivity [ 77 ]. Silver vanadate decorated with silver nanoparticles (AgVO 3 ) is an antimicrobial with the advantage of being stable and not forming agglomerations. When incorporated into acrylic resin at low concentrations (0.5%; 1%; 2.5%; 5%; and 10%) it inhibited the growth of Candida albicans , Streptococcus mutans , Staphylococcus aureus , and Pseudomonas aeruginosa [ 78 , 79 ]. When incorporated into a soft denture liner, 5% was effective against Pseudomonas aeruginosa, Enterococcus faecalis , Candida albicans . However, none of the concentrations tested (1% and 2.5% 5% and 10%) was effective against S. aureus [ 33 ]. AgVO 3 at a concentration of 2.5% incorporated into irreversible hydrocolloid acted as an antimicrobial agent without affecting the physicomechanical properties [ 80 ]. Furthermore, the incorporation of AgVO 3 into endodontic sealers did not induce DNA breaks in human gingival fibroblast or cell death by apoptosis [ 81 ].
The white spots formation is an undesirable side effect of orthodontic therapy [ 82 ], however, it is known that such appliances can affect hygienic ability, alter the oral microflora, and increase levels of acidogenic bacteria such as streptococcus mutans [ 82 ]. The addition of 0.11%, 0.18%, and 0.33% (w/w) AgNP to an orthodontic adhesive (Transbond) reduced the adhesive capacity, on the other hand, growth inhibition of S. mutans was reported after 48 h [ 83 ]. According to Barszczewska-Rybarek & Chladek [ 84 ], the higher the concentration of silver nanoparticles in composites containing Bis-GMA/TEGDMA the lower the degree of conversion, which consequently reduces the adhesive capacity.
Titanium dioxide (TiO 2 ) nanostructures are interesting because of their photocatalytic properties, are non-toxic, inexpensive, and have a high modulus of elasticity (230 GPa) and high refractive index, which allows modulation of the degree of opacity, brightness, and opalescence [ 85 ]. Cao et al. [ 86 ], evaluated brackets coated with a thin film of TiO 2 nanoparticles with nitrogen and reported antimicrobial properties against Streptococcus mutans , Lactobacillus acidophilus , Actinomyces viscous , and Candida albicans . Dias et al., [ 87 ] studied composite resin modified by TiO 2 and TiO 2 /Ag nanoparticles and found that increasing the nanoparticle content reduced bacterial growth. However, high Ag concentrations affect color stability and compromise homogeneous distribution in the composite resin. And yet, Guimarães et al., [ 85 ] observed changes in the degree of conversion and Knoop microhardness when TiO 2 nanostructures functionalized with 3-(aminopropyl) triethoxysilane (APTMS) and 3-(trimethoxysil) propyl methacrylate (TSMPM) were incorporated into a resin. Cibim et al., [ 88 ] observed that adding 5% TiO 2 to a conventional glass ionomer cement significantly increased Knoop microhardness, however, a limitation was obtaining a homogeneous mixture. Garcia-Contreras et al. [ 89 ] reported that low concentrations (3% and 5%) of TiO 2 nanotubes did not interfere with the adhesion of glass ionomer cement to dental tissues. And 5% improved its compressive strength [ 90 ].
Nanohydroxyapatite is another agent studied in order to improve mechanical, morphological, antibacterial, and fluoride release properties of materials such as glass ionomer cement. Alatawi et al., [ 91 ] observed improved compressive strength and antibacterial effect against Streptococcus mutans in addition to increased fluoride ion release. Jardim et al., [ 92 ] observed that dental composites with higher content of hydroxyapatite nanoparticles (HApNP) released higher amounts of Ca 2+ and PO 4 3− and the release rate was pH-dependent, i.e., they released higher amounts at pH 4 and 5.5 than at pH 7. Nano hydroxyapatite doped with strontium showed the formation of agglomerations instead of stable individual particles [ 93 ]. Sodagar et al. [ 94 ], observed that orthodontic adhesive disks containing 5 and 10% silver/hydroxyapatite nanoparticles exhibit antibacterial properties against biofilms, but the same effect was not observed when 1% silver/hydroxyapatite nanoparticles were added to the orthodontic adhesive.
The development of surfaces with bioactive, functionalized coatings, and with controlled release of metallic nanoparticles aims to reduce infection rates. However, concerns over cytotoxicity, bioaccumulation, acquired autoimmunity, and systemic toxicity has gained attention in the same importance status [ 95 ]. Wang et al. [ 96 ], observed a significant antibacterial effect for S. aureus when titanium surfaces were coated with silver nanoparticles, and further, stated that the nanoparticles were safely anchored to the titanium surface in addition to being non-cytotoxic.
Implant bioengineering also studies hybrid strategies that attempt to associate topography with the use of antimicrobial agents. In this sense, different additive manufacturing specimens received the addition of antimicrobial agents, such as silver ions or nanoparticles [ [97] , [98] , [99] ], antibiotic drugs [ 30 , 100 ], the addition of copper or silver to the titanium alloy [ 101 , 102 ], ZnO nanoarrays [ 103 ], modifications by calcium phosphate incorporated into TiO 2 nanotubes [ 104 ] and among others polystyrene and acrylic acid solutions [ 105 ]. Sarker et al. [ 106 ], fabricated additive manufacturing specimens with tilts and observed a reduction in S. aureus biofilm formation, and these results were associated with changes in surface topography, such as wettability and roughness.
Although there are many antibacterial agents applied in order to reduce the biofilm formation, the current context still shows resistant species to antimicrobial therapies, so it is necessary more studies in order to control or reduce pathogenic biofilms looking for new products and techniques. In this sense, the efforts made by materials engineering are essential.
Of the biofilm control strategies that act directly on bacteria, quorum-suppressing enzymes or quorum sensing inhibitors act by inactivating acyl-homoserine lactone molecules (AHLs) which consequently prevents bacteria from synchronizing their virulent behavior [ 10 , 62 , 107 ]. Some examples of these enzymes are lactonase, acylase, oxidoreductase, and paraoxonase. Some plant or animal derivatives are also able to degrade AHL signals and lead to quorum-sensing signal disruption [ 51 , 62 ]. And furthermore, one can inhibit quorum sensing mechanisms that are microorganism-specific, such as the QPS of Pseudomonas aeruginosa , the competence-stimulating peptide, or the X-inducing peptide of S. mutans [ 51 , 71 ], the intercellular adhesive polysaccharide (IAP) produced by S. epidermidis , which is essential for cell-to-cell attachment and subsequent biofilm development, and the autoinducer peptide signal (AIP) synthesized and secreted by S. aureus [ 20 ].
Another effective way to disperse biofilm is to inhibit the signaling pathways of secondary messengers (c-di-GMP, c-di-AMP, and the (p)ppGpp) or reduce the intracellular levels of these messengers [ 48 , 107 ]. This can be achieved by hindering the synthesis of the molecules or by direct degradation/inactivation [ 10 , 51 ]. An example would be the use of small organic molecules that can inactivate messengers such as c-di-GMP, and interfere with biofilm formation in addition to contributing to the destruction of the pre-formed biofilm by inhibiting the synthesis of matrix components [ 8 , 51 ].
Another possible approach is to prevent the matrix from forming. By disintegrating its bonds, it loses its protective effect, leading to biofilm dispersion and the release of planktonic cells. Changes also occur in the gene expression of the bacteria, making them susceptible to antimicrobials [ 8 , 10 ]. Some potential agents are matrix-degrading or biofilm-dispersing enzymes, which may be useful agents for the treatment and prevention of biofilm-related infections in clinical settings [ 108 ]. Some examples of EPS matrix-degrading enzymes include deoxyribonucleases (DNase I, purified human DNase1L2, Bacillus licheniformis extracellular recombinant NucB, Staphylococcus aureus thermonuclease), restriction endonucleases, glycosidic hydrolases, proteases, and dispersin B [ 10 , 51 , 107 , 108 ].
Bacteria use, in addition to quorum sensing, a variety of sensory systems to monitor their environment to enable adaptation to stress conditions [ 48 ]. And in this sense, different strategies are studied to act on these systems. One example is the enzyme Sortase A, present in the cell wall of Gram-positive bacteria [ 56 ], which is responsible for attaching proteins to the envelope surface. Due to the localization of this enzyme, it is studied as a target for anti-virulence drug development [ 56 ]. Small molecule inhibitors may be able to block SrtA by inhibiting the incorporation of surface proteins into the staphylococcal envelope. Thus, they may be useful as anti-infectives to prevent S. aureus infection without affecting the growth of other bacteria, since SrtA inhibitors may interfere with adhesion and intercellular communication rather than bacterial growth [ 109 ]. Another example is proteases, which are degradative enzymes secreted by many bacteria that can interfere with cell-to-cell communication by degrading the competence-stimulating peptide [ 8 ].

8. Conclusions and perspectives
The bacterial cell and the medium in which it develops are extremely dynamic, and the modification of one of them can have an impact on the way they interact with each other. If we can understand the forces and physical interactions that govern this adaptation, as well as bacterial attachment, we could find ways to control unwanted adhesion. This would make it possible to alleviate infections associated with biomaterials, as well as to control the pathogenicity of biofilms.
Although antimicrobials continue to be the main treatment option for bacterial infections, increased drug resistance makes new alternatives necessary to treat infections and fight the spread of multi-resistant bacteria. A possible strategy that is being studied to control adhesion and biofilm formation is interference with the chemical signal of the quorum-sensing system. If it is possible to interfere with communication, bacteria will have difficulties adapting to the environment.
The search for understanding the mechanism of bacterial adhesion to different materials used in oral rehabilitation can bring new perspectives of research aiming at modifying the surfaces and constituents of dental materials, from the knowledge of bacteria action mechanisms. It is still uncertain whether a universal nanostructured substrate will be found, but an in-depth understanding of adhesion will allow technological advances in the medical and dental fields, capable of introducing more effective antimicrobial surfaces.
Conflict of Interes
The authors report no conflicts of interest.
Data availability statement
Available by request.
This research did not receive any specific grant from funding agencies in the public, commercial, or not-for-profit sectors.
- 1. Straub H., Bigger C.M., Valentin J., Abt D., Qin X.H., Eberl L. Bacterial adhesion on Soft materials: passive physicochemical interactions or active bacterial mechanosensing? Adv. Healthc. Mater. 2019;8(8) doi: 10.1002/adhm.201801323. [ DOI ] [ PubMed ] [ Google Scholar ]
- 2. Berne C., Ellison C.K., Ducret A., Brun Y.V. Bacterial adhesion at the single-cell level. Nat Rev Microbiol. 2018;16(10):616–627. doi: 10.1038/s41579-018-0057-5. [ DOI ] [ PubMed ] [ Google Scholar ]
- 3. Alam F., Balani K. Adhesion force of staphylococcus aureus on various biomaterial surfaces. J Mech Behav Biomed Mater. 2017;65:872–880. doi: 10.1016/j.jmbbm.2016.10.009. [ DOI ] [ PubMed ] [ Google Scholar ]
- 4. Han A., Tsoi J.K., Rodrigues F.P., Leprince J.G., Palin W.M. Bacterial adhesion mechanisms on dental implant surfaces and the influencing factors. Int J Adhes Adhes. 2016;69:58–71. doi: 10.1016/j.ijadhadh.2016.03.022. [ DOI ] [ Google Scholar ]
- 5. Schuldt L., Bi J., Owen G., Shen Y., Haapasalo M., Häkkinen L. Decontamination of rough implant surfaces colonized by multispecies oral biofilm by application of leukocyte- and platelet-rich fibrin. J Periodontol. 2020:1–11. doi: 10.1002/JPER.20-0205. [ DOI ] [ PubMed ] [ Google Scholar ]
- 6. Daubert D.M., Weinstein B.F. Biofilm as a risk factor in implant treatment. Periodontology 2000. 2019;81(1):29–40. doi: 10.1111/prd.12280. [ DOI ] [ PubMed ] [ Google Scholar ]
- 7. Dalago H.R., Schuldt Filho G., Rodrigues M.A.P., Renvert S., Bianchini M.A. Risk indicators for peri-implantitis. A cross-sectional study with 916 implants. Clin. Oral Implant Res. 2017;28:144–150. doi: 10.1111/clr.12772. [ DOI ] [ PubMed ] [ Google Scholar ]
- 8. Jakubovics N.S., Goodman S.D., Mashburn-Warren L., Stafford G.P., Cieplik F. The dental plaque biofilm matrix. Periodontology 2000. 2021;86:32–56. doi: 10.1111/prd.12361. [ DOI ] [ PMC free article ] [ PubMed ] [ Google Scholar ]
- 9. Lee S.W., Phillips K.S., Gu H., Kazemzadeh-Narbat M., Ren D. How microbes read the map: effects of implant topography on bacterial adhesion and biofilm formation. Biomatererials. 2021 doi: 10.1016/j.biomaterials.2020.120595. [ DOI ] [ PubMed ] [ Google Scholar ]
- 10. Muhammad M.H., Idris A.L., Fan X., Guo Y., Yu Y., Jin X. Beyond risk: bacterial biofilms and their regulating approaches. Front Microbiol. 2020;11:928. doi: 10.3389/fmicb.2020.00928. [ DOI ] [ PMC free article ] [ PubMed ] [ Google Scholar ]
- 11. Saeki E.K., Kobayashi R.K.T., Nakazato G. Quorum sensing system: target to control the spread of bacterial infections. Microb Pathog. 2020;142 doi: 10.1016/j.micpath.2020.104068. [ DOI ] [ PubMed ] [ Google Scholar ]
- 12. Dufrêne Y.F., Persat A. Mechanomicrobiology: how bacteria sense and respond to forces. Nat Rev Microbiol. 2020;18(4):227–240. doi: 10.1038/s41579-019-0314-2. [ DOI ] [ PubMed ] [ Google Scholar ]
- 13. Elbourne A., Chapman J., Gelmi A., Cozzolino D., Crawford R.J., Truong V.K. Bacterial-nanostructure interactions: the role of cell elasticity and adhesion forces. J Colloid Interface Sci. 2019;546:192–210. doi: 10.1016/j.jcis.2019.03.050. [ DOI ] [ PubMed ] [ Google Scholar ]
- 14. Wang C., Hou J., van der Mei H.C., Busscher H.J., Ren Y. Emergent properties in Streptococcus mutans biofilms are controlled through adhesion force sensing by initial colonizers. MBio. 2019;10(5):10. doi: 10.1128/mBio.01908-19. e01908–19. [ DOI ] [ PMC free article ] [ PubMed ] [ Google Scholar ]
- 15. Ren Y., Wang C., Chen Z., Allan E., van der Mei H.C., Busscher H.J. Emergent heterogeneous microenvironments in biofilms: substratum surface heterogeneity and bacterial adhesion force-sensing. FEMS Microbiol Rev. 2018;42(3):259–272. doi: 10.1093/femsre/fuy001. [ DOI ] [ PubMed ] [ Google Scholar ]
- 16. Tolker-Nielsen T. 2nd ed. ASM Press; Washington: 2015. Biofilm development. Microbial biofilms. [ DOI ] [ Google Scholar ]
- 17. Belas R. Biofilms, flagella, and mechanosensing of surfaces by bacteria. Trends Microbiol. 2014;22(9):517–527. doi: 10.1016/j.tim.2014.05.002. [ DOI ] [ PubMed ] [ Google Scholar ]
- 18. Elias S., Banin E. Multi-species biofilms: living with friendly neighbors. FEMS Microbiol Rev. 2012;36(5):990–1004. doi: 10.1111/j.1574-6976.2012.00325.x. [ DOI ] [ PubMed ] [ Google Scholar ]
- 19. Jakubovics N.S. Intermicrobial interactions as a driver for community composition and stratification of oral biofilms. J Mol Biol. 2015;427(23):3662–3675. doi: 10.1016/j.jmb.2015.09.022. [ DOI ] [ PubMed ] [ Google Scholar ]
- 20. Paluch E., Rewak-Soroczyńska J., Jędrusik I., Mazurkiewicz E., Jermakow K. Prevention of biofilm formation by quorum quenching. Appl Microbiol Biotechnol. 2020;104(5):1871–1881. doi: 10.1007/s00253-020-10349-w. [ DOI ] [ PMC free article ] [ PubMed ] [ Google Scholar ]
- 21. Zhu B., Macleod L.C., Kitten T., Xu P. Streptococcus sanguinis biofilm formation & interaction with oral pathogens. Future Microbiol. 2018;13(08):915–932. doi: 10.2217/fmb-2018-0043. [ DOI ] [ PMC free article ] [ PubMed ] [ Google Scholar ]
- 22. Dhayakaran R., Neethirajan S. Microscopic methods in biofilm research. In: Murthy S., editor. Biofilms: emerging concepts and trends. Narosa Publishers; İndia: 2017. [ Google Scholar ]
- 23. Birarda G., Delneri A., Lagatolla C., Parisse P., Cescutti P., Vaccari L. Multi-technique microscopy investigation on bacterial biofilm matrices: a study on Klebsiella pneumoniae clinical strains. Anal Bioanal Chem. 2019;411:7315–7325. doi: 10.1007/s00216-019-02111-7. [ DOI ] [ PubMed ] [ Google Scholar ]
- 24. Viljoen A., Mignolet J., Viela F., Mathelié-Guinlet M., Dufrêne Y.F. How microbes use force to control adhesion. J Bacteriol. 2020;202(12) doi: 10.1128/JB.00125-20. e00125–20. [ DOI ] [ PMC free article ] [ PubMed ] [ Google Scholar ]
- 25. Goldschmidt G.M., Krok-Borkowicz M., Zybała R., Pamuła E., Telle R., Conrads G. Biomimetic in situ precipitation of calcium phosphate containing silver nanoparticles on zirconia ceramic materials for surface functionalization in terms of antimicrobial and osteoconductive properties. Dent Mater. 2021;37(1):10–18. doi: 10.1016/j.dental.2020.09.018. [ DOI ] [ PubMed ] [ Google Scholar ]
- 26. Jaworska J., Jelonek K., Jaworska‐Kik M., Musiał‐Kulik M., Marcinkowski A., Szewczenko J. Development of antibacterial, ciprofloxacin-eluting biodegradable coatings on Ti6Al7Nb implants to prevent peri-implant infections. J Biomed Mater Res A. 2020;108(4):1006–1015. doi: 10.1002/jbm.a.36877. [ DOI ] [ PubMed ] [ Google Scholar ]
- 27. Bui V.D., Mwangi J.W., Meinshausen A.K., Mueller A.J., Bertrand J., Schubert A. Antibacterial coating of Ti–6Al–4V surfaces using silver nano-powder mixed electrical discharge machining. Surf Coat Technol. 2020;383 doi: 10.1016/j.surfcoat.2019.125254. [ DOI ] [ Google Scholar ]
- 28. Astasov-Frauenhoffer M., Koegel S., Waltimo T., Zimmermann A., Walker C., Hauser-Gerspach I. Antimicrobial efficacy of copper-doped titanium surfaces for dental implants. J Mater Sci Mater Med. 2019;30(7):1–9. doi: 10.1007/s10856-019-6286-y. [ DOI ] [ PubMed ] [ Google Scholar ]
- 29. Li X., Qi M., Sun X., Weir M.D., Tay F.R., Oates T.W. Surface treatments on titanium implants via nanostructured ceria for antibacterial and anti-inflammatory capabilities. Acta Biomater. 2019;94:627–643. doi: 10.1016/j.actbio.2019.06.023. [ DOI ] [ PubMed ] [ Google Scholar ]
- 30. Cox S.C., Jamshidi P., Eisenstein N.M., Webber M.A., Hassanin H., Attallah M.M. Adding functionality with additive manufacturing: fabrication of titanium-based antibiotic eluting implants. Mater Sci Eng C Mater Biol Appl. 2016;64:407–415. doi: 10.1016/j.msec.2016.04.006. [ DOI ] [ PubMed ] [ Google Scholar ]
- 31. De Giglio E., Cafagna D., Cometa S., Allegretta A., Pedico A., Giannossa L.C. An innovative, easily fabricated, silver nanoparticle-based titanium implant coating: development and analytical characterization. Anal Bioanal Chem. 2013;405(2):805–816. doi: 10.1007/s00216-012-6293-z. [ DOI ] [ PubMed ] [ Google Scholar ]
- 32. Holtz R.D., Souza Filho A.G., Brocchi M., Martins D., Durán N., Alves O.L. Development of nanostructured silver vanadates decorated with silver nanoparticles as a novel antibacterial agent. Nanotechnology. 2010;21(18) doi: 10.1088/0957-4484/21/18/185102. [ DOI ] [ PubMed ] [ Google Scholar ]
- 33. Kreve S., Oliveira V.C., Bachmann L., Alves O.L., Dos Reis A.C. Influence of AgVO3 incorporation on antimicrobial properties, hardness, roughness and adhesion of a soft denture liner. Sci Rep. 2019;9(1):1–9. doi: 10.1038/s41598-019-48228-8. [ DOI ] [ PMC free article ] [ PubMed ] [ Google Scholar ]
- 34. Linklater D.P., Juodkazis S., Ivanova E.P. Nanofabrication of mechano-bactericidal surfaces. Nanoscale. 2017;9(43):16564–16585. doi: 10.1039/C7NR05881K. [ DOI ] [ PubMed ] [ Google Scholar ]
- 35. Wu S., Zhang B., Liu Y., Suo X., Li H. Influence of surface topography on bacterial adhesion: a review. Biointerphases. 2018;13(6) doi: 10.1116/1.5054057. [ DOI ] [ PubMed ] [ Google Scholar ]
- 36. Carniello V., Peterson B.W., van der Mei H.C., Busscher H.J. Physico-chemistry from initial bacterial adhesion to surface-programmed biofilm growth. Adv Colloid Interface Sci. 2018;261:1–14. doi: 10.1016/j.cis.2018.10.005. [ DOI ] [ PubMed ] [ Google Scholar ]
- 37. Grzeszczuk Z., Rosillo A., Owens Ó, Bhattacharjee S. Atomic force microscopy (AFM) As a surface mapping tool in microorganisms resistant toward antimicrobials: a mini-review. Front Pharmacol. 2020;11:1588. doi: 10.3389/fphar.2020.517165. [ DOI ] [ PMC free article ] [ PubMed ] [ Google Scholar ]
- 38. Wang C., Hou J., van der Mei H.C., Busscher H.J., Ren Y. Emergent properties in Streptococcus mutans biofilms are controlled through adhesion force sensing by initial colonizers. MBio. 2019;10(5) doi: 10.1128/mBio.01908-19. e01908–19. [ DOI ] [ PMC free article ] [ PubMed ] [ Google Scholar ]
- 39. Milles L.F., Gaub H.E. Extreme mechanical stability in protein complexes. Curr Opin Struct Biol. 2020;60:124–130. doi: 10.1016/j.sbi.2019.11.012. [ DOI ] [ PubMed ] [ Google Scholar ]
- 40. James S.A., Hilal N., Wright C.J. Atomic force microscopy studies of bioprocess engineering surfaces - imaging, interactions and mechanical properties mediating bacterial adhesion. Biotechnol J. 2017;12(7) doi: 10.1002/biot.201600698. [ DOI ] [ PubMed ] [ Google Scholar ]
- 41. Merghni A., Kammoun D., Hentati H., Janel S., Popoff M., Lafont F. Quantification of Staphylococcus aureus adhesion forces on various dental restorative materials using atomic force microscopy. Appl Surf Sci. 2016;379:323–330. doi: 10.1016/j.apsusc.2016.04.072. [ DOI ] [ Google Scholar ]
- 42. Fang J., Wang C., Li Y., Zhao Z., Mei L. Comparison of bacterial adhesion to dental materials of polyethylene terephthalate (PET) and polymethyl methacrylate (PMMA) using atomic force microscopy and scanning electron microscopy. Scanning. 2016;38(6):665–670. doi: 10.1002/sca.21314. [ DOI ] [ PubMed ] [ Google Scholar ]
- 43. Aguayo S., Donos N., Spratt D., Bozec L. Probing the nanoadhesion of Streptococcus sanguinis to titanium implant surfaces by atomic force microscopy. Int J Nanomed. 2016;11:1443–1450. doi: 10.2147/IJN.S100768. [ DOI ] [ PMC free article ] [ PubMed ] [ Google Scholar ]
- 44. Harapanahalli A.K., Younes J.A., Allan E., van der Mei H.C., Busscher H.J. Chemical signals and mechanosensing in bacterial responses to their environment. PLoS Pathog. 2015;11(8):e1005057. doi: 10.1371/journal.ppat.1005057. [ DOI ] [ PMC free article ] [ PubMed ] [ Google Scholar ]
- 45. Gordon V.D., Wang L. Bacterial mechanosensing: the force will be with you, always. J Cell Sci. 2019;132(7) doi: 10.1242/jcs.227694. jcs227694. [ DOI ] [ PMC free article ] [ PubMed ] [ Google Scholar ]
- 46. Geng J., Beloin C., Ghigo J.M., Henry N. Bacteria hold their breath upon surface contact as shown in a strain of Escherichia coli, using dispersed surfaces and flow cytometry analysis. PLoS One. 2014;9(7):e102049. doi: 10.1371/journal.pone.0102049. [ DOI ] [ PMC free article ] [ PubMed ] [ Google Scholar ]
- 47. Quintana I.M., Gibhardt J., Turdiev A., Hammer E., Commichau F.M., Lee V.T. The KupA and KupB proteins of Lactococcus lactis IL1403 are novel c-di-AMP receptor proteins responsible for potassium uptake. J Bacteriol. 2019;201(10) doi: 10.1128/JB.00028-19. e00028–19. [ DOI ] [ PMC free article ] [ PubMed ] [ Google Scholar ]
- 48. Hauryliuk V., Atkinson G.C., Murakami K.S., Tenson T., Gerdes K. Recent functional insights into the role of (p)ppGpp in bacterial physiology. Nat Rev Microbiol. 2015;13:298–309. doi: 10.1038/nrmicro3448. [ DOI ] [ PMC free article ] [ PubMed ] [ Google Scholar ]
- 49. Corrigan R.M., Campeotto I., Jeganathan T., Roelofs K.G., Lee V.T., Gründling A. Systematic identification of conserved bacterial c-di-AMP receptor proteins. Proc Natl Acad Sci USA. 2013;110(22):9084–9089. doi: 10.1073/pnas.1300595110. [ DOI ] [ PMC free article ] [ PubMed ] [ Google Scholar ]
- 50. Syal K., Flentie K., Bhardwaj N., Maiti K., Jayaraman N., Stallings C.L. Synthetic (p)ppGpp analogue is an inhibitor of stringent response in mycobacteria. Antimicrob Agents Chemother. 2017;61(6) doi: 10.1128/AAC.00443-17. e00443-17. [ DOI ] [ PMC free article ] [ PubMed ] [ Google Scholar ]
- 51. Parrino B., Schillaci D., Carnevale I., Giovannetti E., Diana P., Cirrincione G. Synthetic small molecules as anti-biofilm agents in the struggle against antibiotic resistance. Eur J Med Chem. 2019;161:154–178. doi: 10.1016/j.ejmech.2018.10.036. [ DOI ] [ PubMed ] [ Google Scholar ]
- 52. Zheng S., Bawazir M., Dhall A., Kim H.E., He L., Heo J. Implication of surface properties, bacterial motility, and hydrodynamic conditions on bacterial surface sensing and their initial adhesion. Front Bioeng Biotechnol. 2021;9 doi: 10.3389/fbioe.2021.643722. [ DOI ] [ PMC free article ] [ PubMed ] [ Google Scholar ]
- 53. Arciola C.R., Campoccia D., Montanaro L. Implant infections: adhesion, biofilm formation and immune evasion. Nat Rev Microbiol. 2018;16(7):397–409. doi: 10.1038/s41579-018-0019-y. [ DOI ] [ PubMed ] [ Google Scholar ]
- 54. Preethanath R.S., AlNahas N.W., Huraib S.M.B., Al-Balbeesi H.O., Almalik N.K., Dalati M.H.N. Microbiome of dental implants and its clinical aspect. Microb Pathog. 2017;106:20–24. doi: 10.1016/j.micpath.2017.02.009. [ DOI ] [ PubMed ] [ Google Scholar ]
- 55. Dramé I., Formosa-Dague C., Lafforgue C., Chapot-Chartier M.P., Piard J.C., Castelain M. Analysis of homotypic interactions of Lactococcus lactis pili using single-cell force spectroscopy. ACS Appl Mater Interfaces. 2020;12(19):21411–21423. doi: 10.1021/acsami.0c03069. [ DOI ] [ PubMed ] [ Google Scholar ]
- 56. Cascioferro S., Totsika M., Schillaci D. Sortase A: an ideal target for anti-virulence drug development. Microb Pathog. 2014;77:105–112. doi: 10.1016/j.micpath.2014.10.007. [ DOI ] [ PubMed ] [ Google Scholar ]
- 57. Sullan R.M.A., Beaussart A., Tripathi P., Derclaye S., El-Kirat-Chatel S., Li J.K. Single-cell force spectroscopy of pili-mediated adhesion. Nanoscale. 2014;6(2):1134–1143. doi: 10.1039/C3NR05462D. [ DOI ] [ PubMed ] [ Google Scholar ]
- 58. Lauga E. Bacterial hydrodynamics. Annu Rev Fluid Mech. 2016;48:105–130. doi: 10.1146/annurev-fluid-122414-034606. [ DOI ] [ Google Scholar ]
- 59. Gu H., Chen A., Song X., Brasch M.E., Henderson J.H., Ren D. How Escherichia coli lands and forms cell clusters on a surface: a new role of surface topography. Sci Rep. 2016;6:29516. doi: 10.1038/srep29516. [ DOI ] [ PMC free article ] [ PubMed ] [ Google Scholar ]
- 60. Darveau R.P., Curtis M.A. Oral biofilms revisited: a novel host tissue of bacteriological origin. Periodontology 2000. 2021;86(1):8–13. doi: 10.1111/prd.12374. [ DOI ] [ PubMed ] [ Google Scholar ]
- 61. Limoli D.H., Jones C.J., Wozniak D.J. Bacterial extracellular polysaccharides in biofilm formation and function. Microbiol Spectr. 2015;3(3) doi: 10.1128/microbiolspec.MB-0011-2014. [ DOI ] [ PMC free article ] [ PubMed ] [ Google Scholar ]
- 62. Rémy B., Mion S., Plener L., Elias M., Chabrière E., Daudé D. Interference in bacterial quorum sensing: a biopharmaceutical perspective. Front Pharmacol. 2018;9:203. doi: 10.3389/fphar.2018.00203. [ DOI ] [ PMC free article ] [ PubMed ] [ Google Scholar ]
- 63. Palmer R.J., Shah N., Valm A., Paster B., Dewhirst F., Inui T. Interbacterial adhesion networks within early oral biofilms of single human hosts. Appl Environ Microbiol. 2017;83(11) doi: 10.1128/AEM.00407-00417. e00407–17. [ DOI ] [ PMC free article ] [ PubMed ] [ Google Scholar ]
- 64. Rowińska I., Szyperska-Ślaska A., Zariczny P., Pasławski R., Kramkowski K., Kowalczyk P. The influence of diet on oxidative stress and inflammation induced by bacterial biofilms in the human oral cavity. Materials (Basel) 2021;14(6):1444. doi: 10.3390/ma14061444. [ DOI ] [ PMC free article ] [ PubMed ] [ Google Scholar ]
- 65. Joseph S., Curtis M.A. Microbial transitions from health to disease. Periodontology 2000. 2021;86(1):201–209. doi: 10.1111/prd.12377. [ DOI ] [ PubMed ] [ Google Scholar ]
- 66. Kistler J.O., Booth V., Bradshaw D.J., Wade W.G. Bacterial community development in experimental gingivitis. PLoS One. 2013;8(8):e71227. doi: 10.1371/journal.pone.0071227. [ DOI ] [ PMC free article ] [ PubMed ] [ Google Scholar ]
- 67. Pokrowiecki R., Zaręba T., Szaraniec B., Pałka K., Mielczarek A., Menaszek E. In vitro studies of nanosilver-doped titanium implants for oral and maxillofacial surgery. Int J Nanomed. 2017;12:4285. doi: 10.2147/IJN.S131163. [ DOI ] [ PMC free article ] [ PubMed ] [ Google Scholar ]
- 68. Do Nascimento C., Pita M.S., de Souza Santos E., Monesi N., Pedrazzi V. Microbiome of titanium and zirconia dental implants abutments. Dent Mater. 2016;32(1):93–101. doi: 10.1016/j.dental.2015.10.014. [ DOI ] [ PubMed ] [ Google Scholar ]
- 69. Basavaraju M., Sisnity V.S., Palaparthy R., Addanki P.K. Quorum quenching: signal jamming in dental plaque biofilms. J Dent Sci. 2016;11(4):349–352. doi: 10.1016/j.jds.2016.02.002. [ DOI ] [ PMC free article ] [ PubMed ] [ Google Scholar ]
- 70. Sikdar R., Elias M. Quorum quenching enzymes and their effects on virulence, biofilm, and microbiomes: a review of recent advances. Expert Rev Anti Infect Ther. 2020;18(12):1221–1233. doi: 10.1080/14787210.2020.1794815. [ DOI ] [ PMC free article ] [ PubMed ] [ Google Scholar ]
- 71. García-Reyes S., Soberón-Chávez G., Cocotl-Yanez M. The third quorum-sensing system of Pseudomonas aeruginosa: Pseudomonas quinolone signal and the enigmatic PqsE protein. J Med Microbiol. 2020;69(1):25–34. doi: 10.1099/jmm.0.001116. [ DOI ] [ PubMed ] [ Google Scholar ]
- 72. Lu N., Zhang W., Weng Y., Chen X., Cheng Y., Zhou P. Fabrication of PDMS surfaces with micro patterns and the effect of pattern sizes on bacteria adhesion. Food Control. 2016;68:344–351. doi: 10.1016/j.foodcont.2016.04.014. [ DOI ] [ Google Scholar ]
- 73. Vasudevan R., Kennedy A.J., Merritt M., Crocker F.H., Baney R.H. Microscale patterned surfaces reduce bacterial fouling-microscopic and theoretical analysis. Colloids Surf B Biointerfaces. 2014;117:225–232. doi: 10.1016/j.colsurfb.2014.02.037. [ DOI ] [ PubMed ] [ Google Scholar ]
- 74. Perera-Costa D., Bruque J.M., González-Martín M.L., Gómez-García A.C., Vadillo-Rodríguez V. Studying the influence of surface topography on bacterial adhesion using spatially organized microtopographic surface patterns. Langmuir. 2014;30(16):4633–4641. doi: 10.1021/la5001057. [ DOI ] [ PubMed ] [ Google Scholar ]
- 75. Chien H.W., Chen X.Y., Tsai W.P., Lee M. Inhibition of biofilm formation by rough shark skin-patterned surfaces. Colloids Surf B Biointerf. 2020;186 doi: 10.1016/j.colsurfb.2019.110738. [ DOI ] [ PubMed ] [ Google Scholar ]
- 76. Du C., Wang C., Zhang T., Yi X., Liang J., Wang H. Reduced bacterial adhesion on zirconium-based bulk metallic glasses by Femtosecond laser. Nanostruct Proc Inst Mech Eng. 2020;234:387–397. doi: 10.1177/0954411919898011. [ DOI ] [ PubMed ] [ Google Scholar ]
- 77. Bapat R.A., Joshi C.P., Bapat P., Chaubal T.V., Pandurangappa R., Jnanendrappa N. The use of nanoparticles as biomaterials in dentistry. Drug Discov Today. 2019;24(1):85–98. doi: 10.1016/j.drudis.2018.08.012. [ DOI ] [ PubMed ] [ Google Scholar ]
- 78. de Castro D.T., Valente M.L., da Silva C.H., Watanabe E., Siqueira R.L., Schiavon M.A. Evaluation of antibiofilm and mechanical properties of new nanocomposites based on acrylic resins and silver vanadate nanoparticles. Arch Oral Biol. 2016;67:46–53. doi: 10.1016/j.archoralbio.2016.03.002. [ DOI ] [ PubMed ] [ Google Scholar ]
- 79. de Castro D.T., Valente M.L.D.C., Aires C.P., Alves O.L., Dos Reis A.C. Elemental ion release and cytotoxicity of antimicrobial acrylic resins incorporated with nanomaterial. Gerodontology. 2017;34(3):320–325. doi: 10.1111/ger.12267. [ DOI ] [ PubMed ] [ Google Scholar ]
- 80. de Castro D.T., Kreve S., Oliveira V.C., Alves O.L., Dos Reis A.C. Development of an impression material with antimicrobial properties for dental application. J Prosthodont. 2019;28(8):906–912. doi: 10.1111/jopr.13100. [ DOI ] [ PubMed ] [ Google Scholar ]
- 81. Teixeira A.B.V., Moreira N.C.S., Takahashi C.S., Schiavon M.A., Alves O.L., Reis A.C. Cytotoxic and genotoxic effects in human gingival fibroblast and ions release of endodontic sealers incorporated with nanostructured silver vanadate. J Biomed Mater Res B Appl Biomater. 2021:1–10. doi: 10.1002/jbm.b.34798. [ DOI ] [ PubMed ] [ Google Scholar ]
- 82. Borzabadi-Farahani A., Borzabadi E., Lynch E. Nanoparticles in orthodontics, a review of antimicrobial and anti-caries applications. Acta Odontol Scand. 2014;72(6):413–417. doi: 10.3109/00016357.2013.859728. [ DOI ] [ PubMed ] [ Google Scholar ]
- 83. Degrazia F.W., Leitune V.C., Garcia I.M., Arthur R.A., Samuel S.M., Collares F.M. Effect of silver nanoparticles on the physicochemical and antimicrobial properties of an orthodontic adhesive. J Appl Oral Sci. 2016;24(4):404–410. doi: 10.1590/1678-775720160154. [ DOI ] [ PMC free article ] [ PubMed ] [ Google Scholar ]
- 84. Barszczewska-Rybarek I., Chladek G. Studies on the curing efficiency and mechanical properties of Bis-GMA and TEGDMA nanocomposites containing silver nanoparticles. Int J Mol Sci. 2018;19(12):3937. doi: 10.3390/ijms19123937. [ DOI ] [ PMC free article ] [ PubMed ] [ Google Scholar ]
- 85. Guimarães G.M.F., Bronze-Uhle E.S., Lisboa-Filho P.N., Fugolin A.P.P., Borges A.F.S., Gonzaga C.C. Effect of the addition of functionalized TiO2nanotubes and nanoparticles on properties of experimental resin composites. Dent Mater. 2020;36(12):1544–1556. doi: 10.1016/j.dental.2020.09.013. [ DOI ] [ PubMed ] [ Google Scholar ]
- 86. Cao B., Wang Y., Li N., Liu B., Zhang Y. Preparation of an orthodontic bracket coated with an nitrogen-doped TiO(2-x)N(y) thin film and examination of its antimicrobial performance. Dent Mater J. 2013;32(2):311–316. doi: 10.4012/dmj.2012-155. [ DOI ] [ PubMed ] [ Google Scholar ]
- 87. Dias H.B., Bernardi M.I.B., Bauab T.M., Hernandes A.C., de Souza Rastelli A.N. Titanium dioxide and modified titanium dioxide by silver nanoparticles as an anti biofilm filler content for composite resins. Dent Mater. 2019;35(2):e36–e46. doi: 10.1016/j.dental.2018.11.002. [ DOI ] [ PubMed ] [ Google Scholar ]
- 88. Cibim D.D., Saito M.T., Giovani P.A., Borges A.F.S., Pecorari V.G.A., Gomes O.P. Novel nanotechnology of TiO2 improves physical-chemical and biological properties of glass ionomer cement. Int J Biomater. 2017;2017 doi: 10.1155/2017/7123919. [ DOI ] [ PMC free article ] [ PubMed ] [ Google Scholar ]
- 89. Garcia-Contreras R., Scougall-Vilchis R.J., Contreras-Bulnes R., Sakagami H., Morales-Luckie R.A., Nakajima H. Mechanical, antibacterial and bond strength properties of nano-titanium-enriched glass ionomer cement. J Appl Oral Sci. 2015;23(May–June (3)):321–328. doi: 10.1590/1678-775720140496. [ DOI ] [ PMC free article ] [ PubMed ] [ Google Scholar ]
- 90. Kantovitz K.R., Fernandes F.P., Feitosa I.V., Lazzarini M.O., Denucci G.C., Gomes O.P. TiO2 nanotubes improve physico-mechanical properties of glass ionomer cement. Dent Mater. 2020;36(3):e85–e92. doi: 10.1016/j.dental.2020.01.018. [ DOI ] [ PubMed ] [ Google Scholar ]
- 91. Alatawi R.A., Elsayed N.H., Mohamed W.S. Influence of hydroxyapatite nanoparticles on the properties of glass ionomer cement. J Mater Res Technol. 2019;8(1):344–349. doi: 10.1016/j.jmrt.2018.01.010. [ DOI ] [ Google Scholar ]
- 92. Jardim R.N., Rocha A.A., Rossi A.M., de Almeida Neves A., Portela M.B., Lopes R.T. Fabrication and characterization of remineralizing dental composites containing hydroxyapatite nanoparticles. J Mech Behav Biomed Mater. 2020;109 doi: 10.1016/j.jmbbm.2020.103817. [ DOI ] [ PubMed ] [ Google Scholar ]
- 93. Krishnan V., Bhatia A., Varma H. Development, characterization and comparison of two strontium doped nano hydroxyapatite molecules for enamel repair/regeneration. Dent Mater. 2016;32(5):646–659. doi: 10.1016/j.dental.2016.02.002. [ DOI ] [ PubMed ] [ Google Scholar ]
- 94. Sodagar A., Akhavan A., Hashemi E., Arab S., Pourhajibagher M., Sodagar K. Evaluation of the antibacterial activity of a conventional orthodontic composite containing silver/hydroxyapatite nanoparticles. Prog Orthod. 2016;17(1):40. doi: 10.1186/s40510-016-0153-x. [ DOI ] [ PMC free article ] [ PubMed ] [ Google Scholar ]
- 95. Kunrath M.F., Campos M.M. Metallic-nanoparticle release systems for biomedical implant surfaces: effectiveness and safety. Nanotoxicology. 2021;24:1–19. doi: 10.1080/17435390.2021.1915401. [ DOI ] [ PubMed ] [ Google Scholar ]
- 96. Wang G., Jin W., Qasim A.M., Gao A., Peng X., Li W. Antibacterial effects of titanium embedded with silver nanoparticles based on electron-transfer-induced reactive oxygen species. Biomaterials. 2017;124:25–34. doi: 10.1016/j.biomaterials.2017.01.028. [ DOI ] [ PubMed ] [ Google Scholar ]
- 97. van Hengel I.A.J., Riool M., Fratila-Apachitei L.E., Witte-Bouma J., Farrell E., Zadpoor A.A. Selective laser melting porous metallic implants with immobilized silver nanoparticles kill and prevent biofilm formation by methicillin-resistant Staphylococcus aureus. Biomaterials. 2017;140:1–15. doi: 10.1016/j.biomaterials.2017.02.030. [ DOI ] [ PubMed ] [ Google Scholar ]
- 98. Bakhshandeh S., Gorgin Karaji Z., Lietaert K., Fluit A.C., Boel C.H.E., Vogely H.C. Simultaneous delivery of multiple antibacterial agents from additively manufactured porous biomaterials to fully eradicate planktonic and adherent Staphylococcus aureus. ACS Appl Mater Interfaces. 2017;9(31):25691–25699. doi: 10.1021/acsami.7b04950. [ DOI ] [ PMC free article ] [ PubMed ] [ Google Scholar ]
- 99. Amin Yavari S., Loozen L., Paganelli F.L., Bakhshandeh S., Lietaert K., Groot J.A. Antibacterial behavior of additively manufactured porous titanium with nanotubular surfaces releasing silver ions. ACS Appl Mater Interfaces. 2016;8(27):17080–17089. doi: 10.1021/acsami.6b03152. [ DOI ] [ PubMed ] [ Google Scholar ]
- 100. Vaithilingam J., Kilsby S., Goodridge R.D., Christie S.D., Edmondson S., Hague R.J. Immobilisation of an antibacterial drug to Ti6Al4V components fabricated using selective laser melting. Appl Surf Sci. 2014;314:642–654. doi: 10.1016/j.apsusc.2014.06.014. [ DOI ] [ Google Scholar ]
- 101. Macpherson A., Li X., McCormick P., Ren L., Yang K., Sercombe T.B. Antibacterial titanium produced using selective laser melting. JOM. 2017;69(12):2719–2724. doi: 10.1007/s11837-017-2589-y. [ DOI ] [ Google Scholar ]
- 102. Guo S., Lu Y., Wu S., Liu L., He M., Zhao C. Preliminary study on the corrosion resistance, antibacterial activity and cytotoxicity of selective-laser-melted Ti6Al4V-xCu alloys. Mater Sci Eng C Mater Biol Appl. 2017;72:631–640. doi: 10.1016/j.msec.2016.11.126. [ DOI ] [ PubMed ] [ Google Scholar ]
- 103. Xue C., Shi X., Fang X., Tao H., Zhu H., Yu F. The "pure marriage" between 3D printing and well-ordered nanoarrays by using PEALD assisted hydrothermal surface engineering. ACS Appl Mater Interfaces. 2016;8(13):8393–8400. doi: 10.1021/acsami.6b01417. [ DOI ] [ PubMed ] [ Google Scholar ]
- 104. Hu X., Xu R., Yu X., Chen J., Wan S., Ouyang J. Enhanced antibacterial efficacy of selective laser melting titanium surface with nanophase calcium phosphate embedded to TiO2 nanotubes. Biomed Mater. 2018;13(4) doi: 10.1088/1748-605X/aac1a3. [ DOI ] [ PubMed ] [ Google Scholar ]
- 105. Vargas-Alfredo N., Dorronsoro A., Cortajarena A.L., Rodríguez-Hernández J. Antimicrobial 3D porous scaffolds prepared by additive manufacturing and breath figures. ACS Appl Mater Interfaces. 2017;9(42):37454–37462. doi: 10.1021/acsami.7b11947. [ DOI ] [ PubMed ] [ Google Scholar ]
- 106. Sarker A., Tran N., Rifai A., Brandt M., Tran P.A., Leary M. Rational design of additively manufactured Ti6Al4V implants to control Staphylococcus aureus biofilm formation. Materialia. 2019;5 doi: 10.1016/j.mtla.2019.100250. [ DOI ] [ Google Scholar ]
- 107. Sharahi J.Y., Azimi T., Shariati A., Safari H., Tehrani M.K., Hashemi A. Advanced strategies for combating bacterial biofilms. J Cell Physiol. 2019 doi: 10.1002/jcp.28225. [ DOI ] [ PubMed ] [ Google Scholar ]
- 108. Kaplan J.B. Biofilm matrix-degrading enzymes. Methods Mol Biol. 2014;1147:203–213. doi: 10.1007/978-1-4939-0467-9_14. [ DOI ] [ PubMed ] [ Google Scholar ]
- 109. Barthels F., Marincola G., Marciniak T., Konhäuser M., Hammerschmidt S., Bierlmeier J. Asymmetric disulfanylbenzamides as irreversible and selective inhibitors of Staphylococcus aureus Sortase A. ChemMedChem. 2020;15(10):839–850. doi: 10.1002/cmdc.201900687. [ DOI ] [ PMC free article ] [ PubMed ] [ Google Scholar ]
Associated Data
This section collects any data citations, data availability statements, or supplementary materials included in this article.
Data Availability Statement
- View on publisher site
- PDF (1.2 MB)
- Collections
Similar articles
Cited by other articles, links to ncbi databases.
- Download .nbib .nbib
- Format: AMA APA MLA NLM
Add to Collections
An official website of the United States government
Official websites use .gov A .gov website belongs to an official government organization in the United States.
Secure .gov websites use HTTPS A lock ( Lock Locked padlock icon ) or https:// means you've safely connected to the .gov website. Share sensitive information only on official, secure websites.
- Publications
- Account settings
- Advanced Search
- Journal List
In Vitro and In Vivo Model to Study Bacterial Adhesion to the Vessel Wall Under Flow Conditions
Jorien claes, laurens liesenborghs, marleen lox, peter verhamme, thomas vanassche, marijke peetermans.
- Author information
- Article notes
- Copyright and License information
Correspondence to: Jorien Claes at [email protected]
Collection date 2015.
This is an open-access article distributed under the terms of the Creative Commons Attribution-NonCommercial-NoDerivs 3.0 Unported License. To view a copy of this license, visit http://creativecommons.org/licenses/by-nc-nd/3.0/
In order to cause endovascular infections and infective endocarditis, bacteria need to be able to adhere to the vessel wall while being exposed to the shear stress of flowing blood.
To identify the bacterial and host factors that contribute to vascular adhesion of microorganisms, appropriate models that study these interactions under physiological shear conditions are needed. Here, we describe an in vitro flow chamber model that allows to investigate bacterial adhesion to different components of the extracellular matrix or to endothelial cells, and an intravital microscopy model that was developed to directly visualize the initial adhesion of bacteria to the splanchnic circulation in vivo . These methods can be used to identify the bacterial and host factors required for the adhesion of bacteria under flow. We illustrate the relevance of shear stress and the role of von Willebrand factor for the adhesion of Staphylococcus aureus using both the in vitro and in vivo model.
Keywords: Immunology, Issue 100, Shear stress, Staphylococcus aureus , bacteria, adhesion, mesenteric circulation, von Willebrand factor, flow chamber, vascular infection, infective endocarditis, blood vessel, endothelium, subendothelial matrix
Download video stream .
Introduction
To establish endovascular infections, pathogens require a mechanism to adhere to the endothelium, which lines the vessel wall and the inner surface of the heart, and to persist and establish an infection despite being exposed to the shear stress of rapidly flowing blood. The most frequent pathogen causing life-threatening endovascular infections and infective endocarditis is Staphylococcus aureus ( S. aureus ) 1 .
Various bacterial surface-bound adhesive molecules mediate adhesion to host tissue by interacting with extracellular matrix components. These MSCRAMMs (microbial surface components recognizing adhesive matrix molecules) recognize molecules such as fibronectin, fibrinogen, collagen and von Willebrand factor (VWF). MSCRAMMs are important virulence factors of S. aureus and are implicated in the colonization and invasion of the host 2 . Most studies on these virulence factors have been performed in static conditions, and thus may not be representative for human infections where initial adhesion of the bacteria occurs in flowing blood.
In the case of bloodstream infections, bacteria need to overcome the shearing forces of flowing blood in order to attach to the vessel wall. Models that investigate the interaction between bacteria and endothelium or subendothelium under flow conditions are therefore of particular interest.
A recent study showed that the adhesion of S. aureus to blood vessels under shear stress is mediated by VWF 3 . VWF, a shear stress-operational protein, is released from endothelial cells upon activation. Circulating VWF binds to collagen fibers of the exposed subendothelial matrix. Our group reported that the von Willebrand factor-binding protein (vWbp) of S. aureus is crucial for shear-mediated adhesion to VWF 4 .
In this article, we present an in vitro flow chamber model where bacterial adhesion to different components of the extracellular matrix or to endothelial cells can be evaluated. To validate the findings from in vitro data, we have developed an in vivo model that visualizes and quantifies the direct interaction of bacteria with the vessel wall and the formation of bacteria-platelet thrombi in the mesenteric circulation of mice, using real-time intravital vascular microscopy.
Animal experiments were approved by the Ethical Committee of the KU Leuven.
1. Preparing Bacteria for In Vitro Perfusions and In Vivo Experiments
We used S. aureus strain Newman for all experiments described in this manuscript. S. aureus Newman was stored in Brain Heart Infusion (BHI) with 10% glycerol at -80 °C.
Use a sterile loop to scrape the frozen bacteria off and inoculate in 5 ml Tryptic Soy Broth (TSB) O/N at 37 °C (OD 600 >3).
Wash the bacteria by centrifugation (2,600 g, RT, 5 min) and resuspend the bacterial pellet in 5 ml PBS (phosphate buffered saline).
Prepare a 1 mg/ml solution of 5(6)-carboxy-fluorescein N-hydroxysuccinimidyl ester (carboxy-fluorescein) in ethanol. Dilute the 1 mg/ml carboxy-fluorescein solution to 150 µg/ml in laboratory grade water ( e.g., MilliQ water). Protect the tubes from light with aluminum foil and store at -20 °C.
Centrifuge the bacteria (2,600 x g, RT, 5 min). Resuspend the bacterial pellet in 800 µl PBS and add 200 µl (final concentration of 30 µg/ml for perfusion experiments) or 400 µl (final concentration 50 µg/ml for in vivo experiments) of the 150 µg/ml carboxy-fluorescein solution. Protect the tubes from light with aluminum foil and incubate for 30 min at RT on a shaker.
After labeling, block with 6% bovine serum albumin (BSA) solution in PBS.
Dilute bacteria using optical densitometry (OD), an OD 600 of 0.65 for in vitro experiments (corresponding to approximately 3 x 10 8 colony forming units (CFU)/ml for S. aureus ) and an OD 600 of 1.8 for in vivo experiments (corresponding to approximately 1 x 10 9 CFU/ml for S. aureus ) in PBS. Protect the tubes from light with aluminum foil and leave on ice.
2. In Vitro Perfusion Experiments
- Dilute von Willebrand factor (VWF) (Haemate P, stock concentration 2,400 µg/ml) in laboratory grade water (deionized distilled) to a final concentration of 50 µg/ml.
- Dilute collagen in isotonic glucose solution (SKF solution, pH 2.7-2.9, as supplied by the manufacturer) to a final concentration of 160 µg/ml.
- Coat glass coverslips (24 × 50 mm) with VWF or collagen by dropping 200 µl of the coating on parafilm and place the coverslip on top of the droplet. The droplet will spread along the surface of the coverslip.
- Incubate the coverslip in a humidified container for 4 hr at RT. Carefully lift the coverslips from the parafilm with a blunt needle. Mount the coverslip in the bottom part of the flow chamber.
- Coat plastic slips (1-well PCA cell culture chamber, Sarstedt, Germany) with 1 ml of a 1% gelatin solution in PBS and incubate for 30 min at 37°C. Seed human umbilical vein endothelial cells (HUVECs) on the gelatin coated plastic slips and grow them to 70-80% confluency. Mount the plastic slip in the bottom part of the flow chamber.
- Perform in vitro bacterial adhesion studies in a micro-parallel plate flow chamber at a laminar shear stress between 2.5 dyne/cm 2 and 20 dyne/cm 2 to simulate different physiological flow conditions.
- The flow chamber (in-house design) consists of a metal frame and a perfusion chamber made out of plexiglas (poly(methyl) methacrylate (PMMA)). By connecting it to a high-accuracy infusion pump (PHD 2000 Infusion, Harvard Apparatus, USA), we can generate flow rates between 0.0001 µl/min and 220.82 ml/min.
- Connect the tubing to the upper part of the flow chamber and inject medium in the tubing. Gently place the upper part of the flow chamber on top of the bottom part and assemble the flow chamber. Be careful to avoid air bubbles. Inject 1 ml of medium through the chamber to make sure that the chamber is not leaking and to remove excess coating solution. Avoid air bubbles.
- Place the mouse on a thermo-controlled heating pad at 37 °C on a microscope tray. Since this is a terminal procedure, there is no need for strict asceptic procedures. Make an incision near the jugular vein, gently remove the right side of the cervical muscle and isolate the jugular vein from the surrounding tissue.
- Set up the infusion pump and fluorescence microscope. Infusion pump settings depend on the syringe diameter and the desired flow rate (See section 2.4). From now on, work in a dark room.
- Fill a syringe with fluorescently labeled bacteria and connect it to the inlet tube. Avoid air bubbles. Start the infusion pump for 10 min. The infusion time depends on the shear rate and the coating, bacteria and medium used and should represent the steady state of adhesion.
- After 10 min, wash away unbound bacteria by connecting a syringe with PBS to the inlet tube and starting the infusion pump.
- Take at least 15 images or movies at different locations after the wash process. Bacteria are small and potentially difficult to focus on. Prior to the in vitro flow experiment, the appropriate focal plane can be retrieved by placing a drop of fluorescently labeled bacteria on a coverslip and placing the coverslip in the flow chamber. Then, search for the appropriate focal plane and save the settings. NOTE: During the in vitro flow experiment, capturing the images during the washing step (± 5 min after start) ensures that only the signal for adherent bacteria is captured.
- Add 60 µg/ml VWF to the fluorescently labeled bacteria just before starting the perfusion. Fill a syringe with fluorescently labeled bacteria or fluorescently labeled bacteria supplemented with 60 µg/ml VWF and connect it to the inlet tube. Avoid air bubbles. Repeat steps 2.3.5.2 to 2.3.5.3
- Activate the endothelial cells by perfusion with a 0.1 mM solution of the Ca 2+ -ionophore A23187 (stock solution 10 mM dissolved in dimethyl sulfoxide (DMSO)) in DMEM at the same shear rate as the bacterial perfusion for 10 min by perfusion with a 0.1 mM. Repeat steps 2.3.5.2 to 2.3.5.3.
Calculate shear rate and shear stress as follows. Shear rate = 6Q/wh 2 Where: Q: flow rate in ml/min, w: width in cm, h: height in cm Shear stress (τ) = shear rate x viscosity (µ) Where µ: medium: 0.01 dynes x sec/cm 2 , whole blood: 0.04 dynes x sec/cm 2
- Obtain live images using an inverted fluorescence microscope with a black and white camera and develop using imaging software. Use exposure time of 1.5 sec. Take multiple snapshots (at least 15) randomly spread over the coated surface of the flow chamber and save them in the appropriate file format.
- Perform image analysis with ImageJ. Subtract the background to remove smooth continuous backgrounds from the image (Process – Substract Background) and define the threshold to set lower and upper threshold values, segmenting gray scale images into features of interest. Measure the area limited to the threshold.
- Compare bacterial adhesion, expressed as fluorescent area, e.g., using statistical analysis software. Compare the groups using one-way ANOVA or two-tailed Student’s t- test. Report all values as mean ± standard error of the mean (SEM). Consider a p- value of <0.05 significant (* p <0.05; ** p <0.01; *** p <0.001).
3. In Vivo Mesenteric Perfusion Model
- Fast the mouse the night before the experiment in order to limit bowel movement.
- Give a 6-8 week old mouse (C57Bl/6) pre-operative analgesia by a subcutaneous injection of buprenorphine (0.1 mg/kg body weight (BW)) 20-30 min prior to the surgery.
- Anesthetize the mouse by intra-peritoneal injection of ketamine (125 mg/kg BW) and xylazine (12.5 mg/kg BW). Check by pedal reflex. Apply vet ointment to prevent dryness.
- Place the mouse on a thermo-controlled heating pad at 37 °C on a microscope tray. Since this is a terminal procedure, there is no need for strict asceptic procedures. Make an incision near the jugular vein, gently remove the right side of the cervical muscle and isolate the jugular vein from the surrounding tissue.
- Insert a 2 French intravenous catheter into the right jugular vein for infusion of fluorescently labeled bacteria or other solutions. Open the peritoneal cavity via a midline abdominal incision and use cotton swabs to spread the mesenterium and to visualize the mesenteric arteriolar and venular circulation.
- Place the mouse on the right side on a transparent plate and secure the cannula with tape. Use a hot pack to prevent hypothermia. To prevent dehydration of the tissue, drop 500 µl 0.9 % NaCl on the intestines.
- Work in a dark room. Use cotton swabs to immobilize the vessels and visualize them under an inverted microscope.
- Topically apply 5 µl of a 10 mM solution of the Ca 2+ -ionophore A23187 dissolved in DMSO. After 10 sec, inject 100 µl labeled bacteria (see step 1) through the jugular catheter. Take time-lapse images. After the experiment is finished, euthanize the mouse according to institutional approved guidelines.
- Obtain live images using an inverted fluorescence microscope, captured using a black and white camera and developed using any imaging software. Apply automated exposure time and contrast optimization specific to the equipment used.
- Acquire time-lapse images using the ‘Acquisition’ tool in the toolbar (Multidimensional Acquisitions – Time) using 40 cycles of 1,000 images/sec. Save the images in an appropriate image file format.
- Process images using ImageJ analysis software to measure the area of fluorescent signal per image. Define the threshold to set lower and upper threshold values, segmenting gray scale images into features of interest. Identify the region of interest (blood vessel) and measure the area limited to the threshold and the region of interest. Compare bacterial adhesion, expressed as fluorescent area using any statistical or graphing software.
Representative Results
S. aureus adhesion to VWF, subendothelial matrix and endothelial cells is a shear stress dependent phenomenon
To emphasize the role of shear stress in the interaction between S. aureus and VWF, we performed perfusions over VWF coated coverslips at different shear rates (a schematic overview of the in vitro perfusion model is given in Figure 1 . Adhesion of S. aureus to VWF increased with increasing shear rates from 250 sec -1 to 2,000 sec -1 ( Figure 2 ), indicating that high shear forces do not inhibit but reinforce the adhesion of bacteria to VWF.
In order to investigate the contribution of VWF to bacterial adhesion to collagen, the main component of the subendothelial matrix, we perfused fluorescently labeled S. aureus over collagen in the presence or absence of VWF. In the absence of VWF, adhesion of S. aureus to collagen decreased with increasing shear rates. However, when VWF was present in the medium, the adhesion of S. aureus increased with increasing shear rates ( Figure 3 ).
The in vitro flow model also allows us to examine the adhesion of bacteria to endothelial cells under flow. We perfused HUVECs with fluorescently labeled S. aureus at shear rates from 500 to 2,000 sec -1 . Where indicated, HUVECs were activated with a Ca 2+ -ionophore, to cause release of VWF. Endothelial cell activation and the subsequent VWF release, increased adhesion of S. aureus ( Figure 4A ), which formed typical “string-like” patterns of fluorescently labeled bacterial clusters aligned in the direction of the shear force ( Figure 4B ), suggesting the binding of bacteria along a linear-stretched VWF molecule.
Initial in vivo bacterial adhesion in splanchnic veins is mediated by VWF
Since S. aureus is able to adhere to VWF, we used wildtype mice ( Vwf +/+ ) and VWF-deficient mice ( Vwf − / − ) to investigate bacterial adhesion to the activated vessel wall in vivo . Real-time videomicroscopy of splanchnic veins allowed the in vivo visualization of circulating fluorescently labeled S. aureus (Schematic overview of the in vivo perfusion model is represented in Figure 5 ).
After pharmacological activation of the endothelium by the Ca 2+ -ionophore, we observed rapid local accumulation of individual bacteria and aggregates of bacteria to the vessel wall of WT mice (supplemental Videos 1 and 2). Almost no adhesion of bacteria was observed on the activated vessel wall of Vwf -deficient mice (supplemental Video 3) compared with adhesion in WT mice ( Figure 6 ). The absence of VWF decreases the ability of S. aureus to adhere to the activated vessel wall.
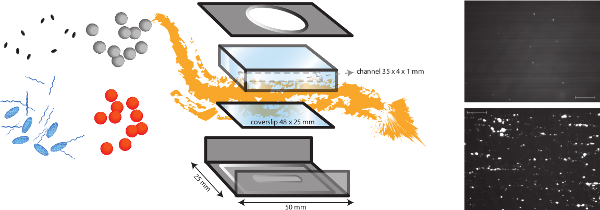
Shear stress is a crucial factor for the early bacterial adhesion to the vessel wall and for the subsequent generation of endovascular or endocardial vegetations and metastatic infections 4,5 . We described complementary in vitro and in vivo models to study the pathogenesis of endovascular infections under physiological shear stress. These models have allowed us to identify von Willebrand factor-binding protein (vWbp) as the major S. aureus protein to interact under flow with an injured vascular wall exposing VWF 4 .
Endovascular infections, and infective endocarditis in particular, are of concern not only because of sepsis-induced organ failure and death, but also because of local and distant (‘metastatic’) complications. To cause infective endocarditis and metastatic infections, bacteria have to adhere to the vessel wall and thus resist the shear stress of flowing blood. Most studies on bacteria virulence factors have been performed in static conditions. However, these established interactions might not withstand shear forces and studies under flow conditions can reveal new, previously unrecognized factors in bacteria-host interplay.
Using the micro-parallel flow chamber, we and others have shown the importance of VWF for vascular adhesion. Under shear stress, VWF progressively unfolds from its resting globular structure, and exposes the A1 domain that interacts with platelets via its GPIb receptor 6 . Flow chambers have been extensively used to study platelet function 7 .
Remarkably, also S. aureus adhesion under flow requires VWF, and in particular the A1 domain that is exposed upon shear. We identified vWbp to mediate VWF binding. vWbp is a coagulase that contributes to S. aureus pathophysiology by activating the host’s prothrombin. Staphylothrombin, the resulting complex of a bacterial coagulase and prothrombin, converts fibrinogen into insoluble fibrin 8,9 . Our studies have shown that vWbp does not only activate prothrombin, but triggers the formation of bacteria-fibrin-platelet aggregates, which enhance the adhesion to blood vessels under flow 4,10,11 .
The in vitro flow chamber model allows to study the different players in bacterial adhesion to cellular or matrix components. Bacterial virulence factors can be studied by using mutants or innocuous bacteria expressing specific surface proteins. Alternatively, pharmacologic inhibitors or blocking antibodies can be added to the medium in the flow chamber. The role of host factors such as different constituents of extracellular matrix can be studied by using coverslips with different coatings. The coverslips can also be covered with endothelial cells, of which the activation status can be modulated by adding specific stimulators. Apart from the vascular wall, the contribution of host blood cells and plasma proteins can be studied by adding these factors to the flowing medium. Thus, different conditions of increasing complexity can be studied under standardized conditions of laminar flow to unravel the interactions that allow bacteria to adhere to the vessel wall in vivo .
Interactions identified in the in vitro model are subsequently studied in an animal model to test their relevance in a complex organism. Other in vivo models to study dynamic interactions under flow have been described, such as the hamster dorsal skinfold chamber 12 and the cremaster model 13 . In comparison, the mesenteric perfusion model described here offers several advantages because of its ease of use, the possibility to vary host genetic background of the mice and to evaluate pharmacological interventions.
In conclusion, the described models offer the possibility to study surface proteins not only of S. aureus , but of many other microorganisms in different host backgrounds, to better understand the pathogenesis of vascular infections.
Disclosures
The authors have nothing to disclose.
Acknowledgments
This work was supported by the Fonds voor Wetenschappelijk Onderzoek (FWO) Vlaanderen G0466.10, 11I0113N; “Eddy Merckx Research Grant” and the “Sporta research Grant” for Pediatric Cardiology, UZ Leuven, Belgium (J.C.); the Center for Molecular and Vascular Biology is supported by the Programmafinanciering KU Leuven (PF/10/014), by the “Geconcentreerde Onderzoeksacties” (GOA 2009/13) from the University of Leuven and a research grant from Boehringer-Ingelheim.
- Vanassche T, Peetermans WE, Herregods MC, Herijgers P, Verhamme P. Anti-thrombotic therapy in infective endocarditis. Expert Rev Cardiovasc Ther. 2011;9(9):1203–1219. doi: 10.1586/erc.11.100. [ DOI ] [ PubMed ] [ Google Scholar ]
- Heying R, van de Gevel J, Que YA, Moreillon P, Beekhuizen H. Fibronectin-binding proteins and clumping factor A in Staphylococcus aureus experimental endocarditis: FnBPA is sufficient to activate human endothelial cells. Thromb Haemost. 2007;97(4):617–626. [ PubMed ] [ Google Scholar ]
- Pappelbaum KI, et al. Ultralarge von Willebrand factor fibers mediate luminal Staphylococcus aureus adhesion to an intact endothelial cell layer under shear stress. Circulation. 2013;128(1):50–59. doi: 10.1161/CIRCULATIONAHA.113.002008. [ DOI ] [ PubMed ] [ Google Scholar ]
- Claes J, et al. Adhesion of Staphylococcus aureus to the vessel wall under flow is mediated by von Willebrand factor–binding protein. Blood. 2014;124(10):1669–1976. doi: 10.1182/blood-2014-02-558890. [ DOI ] [ PMC free article ] [ PubMed ] [ Google Scholar ]
- Thiene G, Basso C. Pathology and pathogenesis of infective endocarditis in native heart valves. Cardiovasc Pathol. 2006;15(5):256–263. doi: 10.1016/j.carpath.2006.05.009. [ DOI ] [ PubMed ] [ Google Scholar ]
- Sixma JJ, Schiphorst ME, Verweij CL, Pannekoek H. Effect of deletion of the A1 domain of von Willebrand factor on its binding to heparin, collagen and platelets in the presence of ristocetin. Eur J Biochem/FEBS. 1991;196(2):369–375. doi: 10.1111/j.1432-1033.1991.tb15826.x. [ DOI ] [ PubMed ] [ Google Scholar ]
- Theilmeier G, Lenaerts T, Remacle C, Collen D, Vermylen J, Hoylaerts MF. Circulating activated platelets assist THP-1 monocytoid/endothelial cell interaction under shear stress. Blood. 1999;94(8):2725–2734. [ PubMed ] [ Google Scholar ]
- Bjerketorp J, Jacobsson K, Frykberg L. The von Willebrand factor-binding protein (vWbp) of Staphylococcus aureus is a coagulase. FEMS Microbiol Lett. 2004;234(2):309–314. doi: 10.1016/j.femsle.2004.03.040. [ DOI ] [ PubMed ] [ Google Scholar ]
- Friedrich R, et al. Staphylocoagulase is a prototype for the mechanism of cofactor-induced zymogen activation. Nature. 2003;425(6957):535–539. doi: 10.1038/nature01962. [ DOI ] [ PubMed ] [ Google Scholar ]
- Vanassche T, et al. Fibrin formation by staphylothrombin facilitates Staphylococcus aureus-induced platelet aggregation. Thromb Haemost. 2012;107(6):1107–1121. doi: 10.1160/TH11-12-0891. [ DOI ] [ PubMed ] [ Google Scholar ]
- Vanassche T, et al. The role of staphylothrombin-mediated fibrin deposition in catheter-related Staphylococcus aureus infections. J Infect Dis. 2013;208(1):92–100. doi: 10.1093/infdis/jit130. [ DOI ] [ PMC free article ] [ PubMed ] [ Google Scholar ]
- Buerkle MA, Lehrer S, Sohn HY, Conzen P, Pohl U, Krötz F. Selective inhibition of cyclooxygenase-2 enhances platelet adhesion in hamster arterioles in vivo. Circulation. 2004;110(14):2053–2059. doi: 10.1161/01.CIR.0000143234.51796.A9. [ DOI ] [ PubMed ] [ Google Scholar ]
- Kim KH, Barazia A, Cho J. Real-time imaging of heterotypic platelet-neutrophil interactions on the activated endothelium during vascular inflammation and thrombus formation in live mice. J Vis Exp. 2013;2(74) doi: 10.3791/50329. [ DOI ] [ PMC free article ] [ PubMed ] [ Google Scholar ]
- View on publisher site
- PDF (526.3 KB)
- Collections
Similar articles
Cited by other articles, links to ncbi databases.
- Download .nbib .nbib
- Format: AMA APA MLA NLM
Add to Collections

IMAGES
COMMENTS
In order to detect the change of bacterial adhesion property of the material after different treatments (e.g., surfactant treatment or material modification) using bioluminescence detection, the sample to be tested was treated with surfactant Triton X-100 before the experiment to reduce the adhesion of bacteria to the material. LB liquid media ...
Feb 15, 2010 · Bacterial adhesion is the initial step in colonization and biofilm formation. Biofilms can, on the one hand, be detrimental to both human life and industrial processes, for example, causing infection, pathogen contamination, and slime formation, while on the other hand, be beneficial in environmental technologies and bioprocesses.
Feb 1, 2005 · Adhesion assay was done as described in 2.4.1 Adhesion of radioactively labelled bacteria, 2.4.2 Adhesion of fluorescent-tagged bacteria—measurement by fluorometer. Then, wells were washed with 250 μl of HEPES–Hanks buffer and the fluorescence from the wells was determined by using Victor 2 1420 Multilabel counter (PerkinElmer).
Jul 26, 2023 · Preparation of bacteria for adherence experiments. Before each experiment, bacteria were cultivated in 6 ml of M9 medium complemented with 0.4% glucose and 200 μg/ml Carbenicillin (all from Merc-Sigma, Israel) in 18 mm glass test tubes for 18 h at 37°C, shaking at 120 round per minute (RPM).
For example, Harris et al. 22 uses the static adhesion assay to assess the effect of surface topography on bacterial adhesion between different orthopedic materials and found that under the conditions of the assay, a smooth surface can result in a reduction in bacterial adhesion for some materials in comparison with microrough equivalents. The ...
Bacterial Adhesion; Tissue Culture Cell; Adherent Bacterium; Positive Cooperativity; Adhesion Experiment; These keywords were added by machine and not by the authors. This process is experimental and the keywords may be updated as the learning algorithm improves.
The adhesion strength of bacteria to certain surfaces is 106–108 times greater than its gravitational force, so it is not surprising that bacteria die from damage to the cell wall as a result of adhesion strength experiments [14,15].
Mass Transport. For a proper analysis of adhesion data, kinetic and stationary or equilibrium effects must be distinguished. Typical kinetics of any bacterial adhesion experiment follows a pattern as shown in Fig. Fig.1, 1, where an initial linear trajectory can be seen, followed by a leveling off to a (pseudo-) end stage.
Jun 11, 2015 · Resuspend the bacterial pellet in 800 µl PBS and add 200 µl (final concentration of 30 µg/ml for perfusion experiments) or 400 µl (final concentration 50 µg/ml for in vivo experiments) of the 150 µg/ml carboxy-fluorescein solution. Protect the tubes from light with aluminum foil and incubate for 30 min at RT on a shaker.
Feb 24, 2006 · Successful establishment of infection by bacterial pathogens requires adhesion to host cells, colonization of tissues, and in certain cases, cellular invasion—followed by intracellular multiplication, dissemination to other tissues, or persistence. Bacteria use monomeric adhesins/invasins or highly sophisticated macromolecular machines such as type III secretion systems and retractile type ...